Chapter 2: Harnessing Biology's Magic
Life scientists yearn to understand the natural world. They persist in trying to unravel the mysteries of biology because these mysteries are inherently intriguing. But more pressingly, scientists want to learn how biological systems work so that they can control them. Such control yields benefits to health and to the human enterprise in countless ways. Harnessing biology's magic underlies the extraordinary promise of biotechnology.
It's Biology, It's Chemistry... It's Engineering!
Metabolic engineering, that is. In this exciting field, biology and chemistry collide head-on. The goal is to use the tools of biology to produce chemical compounds that, in many cases, never before existed in nature: "unnatural" natural products. Metabolic engineers are in the business of using living systems to turn simple sugars and other small molecules into promising new antibiotic drugs—even agricultural and veterinary products.
Some metabolic engineers aren't even engineers, but rather biologists who picked up the requisite chemistry knowledge or chemists who learned the necessary biology. Many metabolic engineers actually are engineers by training. Whatever their background, the common thread is that metabolic engineers think about metabolism with an unconventional pair of eyes. These scientists see metabolic pathways as mini-chemical laboratories, capable of carrying out multiple chemical reactions in a single pot—the cell—without the need for time- and labor-intensive separation and purification steps.
Metabolic engineers work their magic by altering the metabolism of a bacterium, plant, or animal cell. To accomplish such a goal, the researchers must first pick apart the biochemical circuits these organisms routinely use to break down food and produce energy from it (called catabolic pathways), as well as those pathways that re-use the building blocks to make bigger molecules (called anabolic pathways). Metabolic pathways are those involved in the production or breakdown of aptly named molecules called metabolites.
More than a hundred metabolites currently represent mainstay medicines for people, as well as for animals. The antibiotics erythromycin and tetracycline, a cholesterol-lowering drug called lovastatin (Mevacor®), and a flea-busting pet medicine called avermectin are all "polyketides," a class of metabolites that soil bacteria manufacture naturally and abundantly. Since polyketides are made by a chain reaction of many enzymes, metabolic engineers experiment with mixing and matching the genes that encode these enzymes to purposefully alter the ultimate outcome of the series of reactions. By doing so, they can come up with entirely new molecules. Companies have been built upon this prospect, and they are mining nature for the encrypted secrets that can enable microbes to yield medicines that are more powerful or have fewer side effects than those currently in use. Because these methods rely heavily on biology, they are in many cases considerably less toxic than traditional commercial production schemes, which often require the use of potentially hazardous chemicals to yield synthetic compounds.
Metabolic Factories Make Lots From Very Little
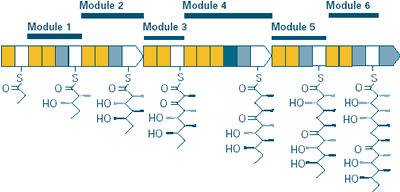
ADAPTED WITH PERMISSION FROM KOSAN BIOSCIENCES
Certain types of soil bacteria make the antibiotic erythromycin—a complicated chemical structure containing 37 atoms of carbon, 13 atoms of oxygen, 67 atoms of hydrogen, and a single atom of nitrogen—beginning with only a couple of small and simple starting materials. The bacteria make this widely used antibiotic through a pathway consisting of a series of enzyme "modules," or mini-factories, each of which is itself made up of at least three different enzymes. In the case of erythromycin production, the job of each module is to progressively add two carbons to the growing chain of atoms destined to become erythromycin.
Combinations, Combinations
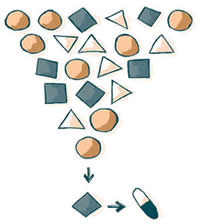
Drug companies large and small are in the business of locating new compounds, called leads, to test for their usefulness in treating disease. In general, the road to drug discovery involves two sequential steps: lead discovery and lead optimization. One popular method some companies use to discover could-be drugs is combinatorial chemistry, a process in which chemists create and then sift through immense collections of compounds ("libraries"). Such screening libraries consist of a diverse, randomly assembled array of thousands of different molecules made from a few starting chemical building blocks. While such approaches can create a bewildering number of possibilities, the lead molecules that are identified through such a scheme may require extensive laboratory tinkering to transform them into body-friendly molecules that work well as drugs.
Endless Possibilities
One of the most challenging aspects of the search for new drugs is combing through reams of possibilities—finding that perfect needle in a towering haystack. An enormous number of new molecules can be generated by a variety of means, but the tough part is finding the good ones. A promising approach chemists are using to develop potential new drugs is combinatorial chemistry, a sort of "needle-finding" technique in which chemists generate breathtaking numbers (millions) of compounds and then attach each one to a miniature plastic bead. The result is an extensive collection, or a library, of many beads, each with a unique molecule attached. Each bead, then, holds a potentially good drug—a needle in a huge haystack of possibilities. Scientists need to be creative in finding ways to distinguish molecules that look very similar to each other but that might act in very different ways. Often, chemists do this by color-coding the process—the best molecule advertises itself by turning red or blue, for instance, when analyzed by a drug-target chemical detection system.
Microbes Go Blue
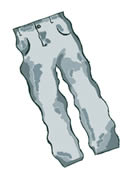
Tooling away in his microbiology lab studying how the bacterium Escherichia coli (E. coli, a common microbe) eats chemicals called hydrocarbons, David Gibson of the University of Texas at Austin noticed a surprising thing: Some of the bacterial spots in his petri dishes had turned a brilliant blue. Looking in his notebook, Gibson realized that those particular specimens were the very ones that he had just spruced up with a new gene—one that coded for an enzyme called toluene dioxygenase. He carefully analyzed the blue pigment produced by the new strain of bacteria and reasoned that the color had been produced when the enzyme converted a simple nutrient called indole into indigo, the world's largest-selling dye and the one that makes blue jeans blue. Coincidentally, the year was 1983, exactly 100 years after 19th-century chemists had devised a method to chemically synthesize the dye, but one that relies on the use of hazardous starting materials (cyanide, for one) and that also generates toxic byproducts. Scientists at Amgen, a company in Thousand Oaks, California, discovered that a very similar enzyme, called napthalene dioxygenase, also turns indole into indigo. Genencor International of Palo Alto, California tried to introduce the biological method to the $200 million indigo market, but ran into trouble. Despite the biotechnical innovation and environmentally friendly nature of getting bacteria to make indigo, as it turns out, the chemical synthesis of this dye is far less expensive, quashing commercial schemes to produce indigo biologically.
Chemistry for a Greener World
While strategies like metabolic engineering offer great promise for spawning new medicines, other areas of chemistry aim to clean up the Earth, improving people's health by ridding the air and waters of pollutants.
Terrence Collins of Carnegie Mellon University in Pittsburgh has developed a class of molecules, called oxidation catalysts, that reduce the amount of chlorine required for industrial bleaching processes. The catalysts, crafted from nontoxic components, may find use in the laundry and paper industries and could also someday be used to treat drinking water by cleansing it of harmful parasites. Collins' catalysts work by jump-starting a natural whitening agent (hydrogen peroxide) that is either present in, or can be easily added to, water-based solutions. A key plus to these green catalysts is that their shelf life can be pre-set. This feature, something Collins calls "dial-a-lifetime," permits scientists to control how long the catalysts stick around before they self-destruct.
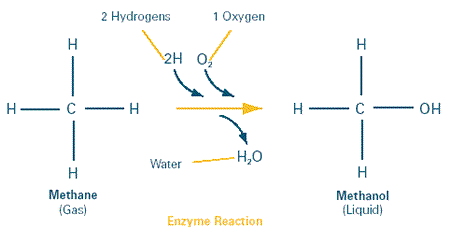
Innovative chemical strategies can help reduce pollution from manufacturing processes, but they might also help mop up after environmental damage has already occurred. To chemists, breaking down small, stubbornly inert molecules such as methane—a single carbon atom framed by four hydrogens—is a technical tour de force. But to a certain variety of bacteria that dwell in hot springs in Bath, England, chewing up methane is a breeze. As miniature apprentices, the hot springs bacteria can provide a valuable service to scientists working to clear the air of methane, which is second only to carbon dioxide as a contributor to the "greenhouse effect" that has been implicated as one source of global warming. In the United States, the most significant sources of methane gas are landfills and agricultural livestock manure.
Researchers and biotechnologists would love to be able to use environmentally friendly biological catalysts to convert methane into methanol. Chemist Stephen Lippard of the Massachusetts Institute of Technology in Cambridge has made major strides in this area by intensively studying the hot springs bacteria to find the magic ingredient—an enzyme—that they use to eat methane.
Lippard and his collaborators figured out what this enzyme, called methane monooxygenase (MMO), looks like and how it likely works. As it turns out, MMO is a curiously complicated molecular machine made up of three separate parts. Together, the three parts manage to transform the tiny methane molecule into methanol in a reaction with oxygen that replaces one hydrogen atom of methane with an oxygen and a hydrogen. This chemical reaction makes methanol, with water as a byproduct (see drawing/illustration above). The fact that a bacterial enzyme can do this is important. While methane is stubbornly difficult to break apart in the laboratory, its chemical cousin methanol can be processed rather easily. Moreover, methanol (a liquid) can be transported much more easily than methane, which is a gas that is harder to keep contained.
Speeding Along
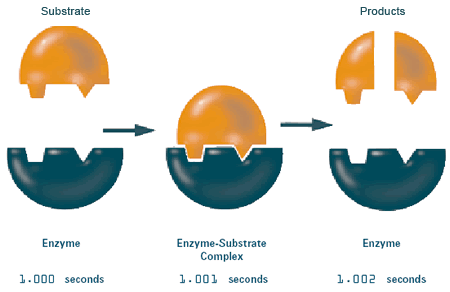
All chemical reactions involve the conversion of starting materials (called substrates) into products. Catalysts are molecules that make reactions go much faster than they would on their own. By definition, catalysts are facilitators—they themselves are not used up in the reaction and can be re-used. In industrial reactions—say, the manufacture of ammonia—catalysts can be a mix of simple molecules such as iron, potassium, oxygen, and aluminum. Your body's catalysts are enzymes. Some enzymes make the reactions taking place in your body occur up to a trillion times faster than they would without any help! Enzymes are therefore essential to life, since your body cannot afford to wait days, weeks, or years to receive the important products of biochemical reactions.
Tiny Bits of Help
Many other scientists are finding ways to use the bacterial work force. Some chemists are using enzymes from bacteria to break down agents of biological warfare. Sarin, the deadly nerve gas sprayed in 1995 into the Tokyo subway system by the cult Aum Shinrikyo, is one example. One of a class of molecules called organophosphates, sarin trips vital nerve circuitry in the bodies of people or animals who come in contact with it; very small quantities can kill a sarin-exposed victim in as little as 5 minutes.
Fortunately, however, certain types of bacteria manufacture an enzyme called phosphotriesterase (PTE) that inactivates sarin and other organophosphate molecules like it, some of which are found in certain insecticides but are hundreds of times less toxic to people. Certain organophosphates, such as the common insecticide malathion, kill insects because, unlike animals, bugs lack an enzyme that breaks down this chemical. For many years Frank Raushel of Texas A&M University in College Station has studied the PTE enzyme, and recently he and his colleague Hazel Holden of the University of Wisconsin-Madison cleared a substantial hurdle: They identified the three-dimensional structure— a "molecular snapshot"—of what this enzyme looks like. This information will help scientists understand how the enzyme works—and could reveal how to engineer one that works even better.
Metals Can Be Good for You...
Enzymes like PTE rely upon a special kind of molecular assistance to do their jobs efficiently. Metals such as iron, zinc, and copper all perform vital roles in some of the enzymatic reactions that fuel the body's metabolism. Iron, for instance, helps the protein hemoglobin transport oxygen to organs in the body. Many metals act to stabilize the shapes of enzymes. But the body's handling of metals—some of which can be highly toxic in excessive amounts—is a tricky business. In some cases, cells exert exquisitely tight control, assuring that only one or two free metal atoms are present inside an individual cell.
Copper is a case in point. Crab and lobster are more than perennial summer favorites—they are also a good dietary source of this metal. Yes, the stuff of pennies is crucial for life—copper is an important helper to many cellular enzymes, including one called superoxide dismutase (SOD), which sops up dangerous "free radicals" that accumulate inside cells. Defects in SOD have been linked to some inherited forms of amyotrophic lateral sclerosis (ALS), also known as Lou Gehrig's disease. Although copper is necessary for life, it is a potentially toxic substance that—in the wrong cellular locale—can damage other molecules, and in some cases even cause disease. A team of scientists including Valeria Culotta of the Johns Hopkins Bloomberg School of Public Health in Baltimore and Thomas O'Halloran and Amy Rosenzweig, both of Northwestern University in Evanston, Illinois, deciphered the three-dimensional structure of a yeast copper "chaperone" protein. This protein transports copper to the SOD enzyme and protects the metal from unwanted cellular interactions along the way. In ALS, researchers suspect, defective SOD—when energized with the copper it needs to function—runs amuck and causes cellular damage. Understanding more about how the copper chaperone—SOD duo trades copper will offer drug designers a crystal—clear glimpse of how to cripple this molecular embrace in patients with Lou Gehrig's disease.
An Electronic Tongue
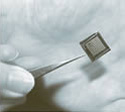
MARSHA MILLER
It's a far cry from the real thing, but a new artificial tongue promises to do a lot more than taste hamburgers and lick stamps. Chemist Eric Anslyn of the University of Texas at Austin and his coworkers have developed a prototype "tongue" biosensor for sampling liquids: a tiny silicon wafer embedded with detection beads that turn color in the presence of specific chemicals. Anslyn's original electronic tongue could only differentiate between acids, simple sugars, and certain electrically charged molecules. In theory, though, many more chemical micro-detectors could be added to the chip, enabling the device to pick out several different components of a solution poured over it. Indeed, an electronic tongue might be a really good thing, considering some of the solutions it might be used to "taste"—blood, urine, and polluted water, for instance. Of course, the system might also be used to assure quality control in much more palatable substances, such as soft drinks.
...or Metals Can Be Bad for You
While it's clear that the body would not work properly without a prescribed amount of a select group of metals, some metals are indeed toxic to the body. Even for the metals your body needs, too much is not a good thing. Since metals are elements (the building blocks of all chemical compounds), as basic components of the Earth they cannot be broken down (like proteins and fats can, for instance). Thus your body takes great care to shepherd these potentially toxic materials to their proper destinations inside cells. As with copper, many other metals are escorted around by protective, chaperone-like molecules.
Some toxic metals, in contrast, aren't good in any amount. They can hinder important enzymes, preventing them from doing their jobs. Lead from the environment, for instance, can trip up the body's synthesis of a vital component of hemoglobin called heme, disabling the blood's oxygen transport system. Some metals can get trapped inside cellular compartments called organelles and pose trouble for normal cell functioning. Certain forms of mercury can be deadly, causing irreversible damage in the brain. Scientists think that some highly toxic metals, such as arsenic, can also cause cancer in the skin and lungs.
Metals in Health and Disease
METAL (Chemical Symbol) | WHERE IS IT? | WHAT DOES IT DO? | HOW DO I GET IT? |
---|---|---|---|
"Healthy" Metals | |||
Iron (Fe) | Binds to enzymes throughout the body (e. g., hemoglobin, nitric oxide synthase) | Helps body transport oxygen and certain chemical messengers | Meats (highest in beef, pork, liver), baked or lima beans, molasses, spinach |
Copper (Cu) | Binds to enzymes throughout the body (e. g., superoxide dismutase) | Defends body against damage from free radicals | Shellfish (crab, lobster), dried beans, nuts |
Zinc (Zn) | Binds to enzymes throughout the body, to DNA, and to some hormones (e. g., insulin) | Plays role in sexual maturation and regulation of hormones, helps some proteins stick tightly to DNA | Shellfish (oysters), chick peas, baked beans, whole grains, nuts |
Sodium (Na) Potassium (K) |
Throughout the body (Na outside cells, K inside cells) | Helps communicate electrical signals in nerves, heart | Na: Table salt and baking soda K: Bananas, oranges, avocados |
Calcium (Ca) | Bones, muscle | Muscle and nerve function; blood clotting | Dairy products, broccoli, figs, sardines |
Cobalt (Co) | Forms the core of vitamin B12 | Necessary ingredient for making red blood cells | Meats, dairy products, leafy green vegetables |
"Unhealthy" Metals | |||
Arsenic (As) | Rocks, soil | Can cause cancer, death | Toxic |
Lead (Pb) | Old paint (before 1973) | Can cause cancer, neurological damage, death | Toxic |
Mercury (Hg) | Contaminated fish (especially from the Great Lakes region of the United States) | Binds to sulfur-containing molecules in organelles; can cause neurological damage, death | Toxic |
Question Box: Future Factories
The Chemistry of Health asked Mary Lidstrom, Professor of Chemical Engineering and Microbiology at the University of Washington in Seattle, "What's new for you on the biotechnology horizon?" Bioremediation isn't necessarily her pressing interest, says Lidstrom, explaining that engineers at companies are well on their way to getting bacteria—especially the kind she studies—to clean up the environment. To her, the real excitement is in getting bacteria to manufacture novel materials cheaply and efficiently, using nontoxic starting materials and generating nontoxic waste products. In her own lab, Lidstrom spends much of her time trying to convince bacteria to do things they weren't meant to do.
And bacteria— they have such amazing metabolism. They can do almost anything.
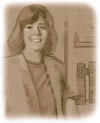
CH: What does the future hold for the kind of biotechnology you're working on?
Lidstrom: The greatest challenge is for the chemical industry to move away from petrochemicals. Our goal is to turn bacteria into chemical factories.
CH: How would that help matters?
Lidstrom: You could use simple sugars like glucose, or other potentially renewable resources— such as methanol—as starting materials.
CH: What challenges do biotechnologists working in this area face?
Lidstrom: The problem is that we can't do this economically right now—the challenge is how to redesign these bacteria. Nature didn't make them to do this.
CH: What turned you on to science?
Lidstrom: I grew up on a cattle ranch in Oregon, where I was around biology all the time. I have always been fascinated with how things work and why—how does light shining on a leaf open it up? And I had great science teachers to nurture my interests.
CH: Why microbiology?
Lidstrom: There's no blood! And bacteria— they have such amazing metabolism. They can do almost anything.
Got It?
What are the substrates used by the enzyme methane monooxygenase? What products does this enzyme make?
What advantages do combinatorial chemistry methods have over the more traditional ways chemists have used to screen for new drugs?
Name an antibiotic manufactured by soil bacteria.
Describe two ways your body uses metals to function properly.
Discuss some of the plusses to using living organisms to produce medicines or other consumer products.