Supernovas Explode in 3D Detail at Oak Ridge National Laboratory
Tags: Astrophysics, Jaguar, Petascale, University of California-Santa Cruz

The inner 1,000 kilometer radius of a 1,700 kilometer radius white dwarf star is simulated using the MAESTRO code on the Jaguar supercomputer. The yellow/green/purple contours show the progression of nuclear energy generation. The red and blue contours indicate regions of outward and inward convection. Image courtesy M. Zingale, Stony Brook University, and A. Nonaka, Lawrence Berkeley National Laboratory
Understanding a Type Ia supernova—an exploding white dwarf star—requires supercomputers. A team of astrophysicists and computational scientists is using the power of Oak Ridge National Laboratory’s (ORNL’s) Jaguar to virtually blow up these white dwarfs. In the process the researchers are revealing the secrets of the biggest thermonuclear explosions in the universe and finding the answers needed to measure the size of the universe.
“The physics of supernova explosions is something astrophysicists have been trying to figure out for about 50 years now,” said Stan Woosley, principal investigator of an Innovative and Novel Computational Impact on Theory and Experiment (INCITE) project and professor of astrophysics at the University of California–Santa Cruz. “It is an interesting physics problem in turbulent combustion, but it is also important because—as the 2011 Nobel Prize attested—Type Ia supernovae can be used to show that the expansion of the universe is accelerating.” This is because the supernovas function as “standard candles,” brilliant lights of known properties usable as measuring tools because their distance can be inferred by how bright they appear.
Using an empirical correlation between the peak luminosity of Type Ia supernovas and the rate at which their brightness declines, three Nobel Prize winners—Saul Perlmutter, Adam Reiss, and Brian Schmidt—determined that distant galaxies were farther away than expected for a universe expanding at a constant rate. Their work showed that the expansion of the universe is, in fact, accelerating and refuted a long-held belief that the universe was expanding at a constant rate or even slowing down. It is as if a tennis ball, thrown straight up, did not fall back to Earth but continued to move away faster and faster. The driver behind this acceleration, called dark energy, is a force not yet fully understood that counters the pull of gravity on matter.
Woosley said his team seeks to understand the nature of the supernovas, find other ways of predicting the supernova’s peak luminosity, and explain the empirical relation between brightness and decline rate (the ratio of maximum light declining over time). Even with the world’s most powerful telescopes, the data obtained through observations is limited and sometimes corrupted by the effects of interstellar dust or the particular characteristics of the telescopes used. Given a valid physical description, the supercomputer can model the supernova without these observational biases. The simulations not only give the supernova’s light curve (the total intensity of light over time) and spectrum (the intensity of light at certain frequencies) at all angles over time, but also allow researchers to look for other measurable quantities that might correlate with peak luminosity.
Presently cosmologists use Type Ia supernovas to measure distances to about 10 percent accuracy. The immediate goal is to reduce the error below 5 percent. To do that, they need a better yardstick, and numerical experiments on the supercomputer are a way to build one.
A second life
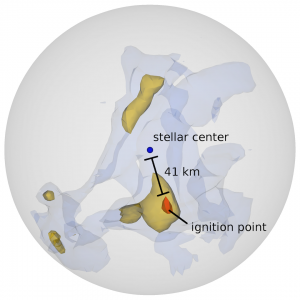
The temperature at the ignition point (red) as thermonuclear runaway ignites is more than 800 million degrees (Kelvin). The blue and gold areas indicate regions where the temperature is greater than 730 million K and 760 million K, respectively. The dark blue dot is at the center of the star, and has a diameter of 4.34 km, which corresponds to the grid cell width for this simulation. Image courtesy M. Zingale, Stony Brook University, and A. Nonaka, Lawrence Berkeley National Laboratory
A white dwarf star begins, as do all stars, when gravity compresses a cloud of helium, hydrogen, and dust. As the density increases, so does the force of gravity. The temperature and density are highest at the center of the cloud. The pressure of the compaction increases the internal temperature. As the pressure and temperature climb, the compaction and heat set off the star’s core fusion reaction. The star begins to glow. After shining 10 billion years or so (for a star of our sun’s mass), its fuel is spent and the star—cooling, fading, and dwindling to the size of Earth—becomes a white dwarf.
With its own energy depleted, the star will continue cooling into a cold, black dwarf unless it can accrete mass from a nearby star.
The white dwarf can accumulate mass in two ways. It can steal mass slowly, from a younger, vibrant companion star still undergoing nuclear fusion until the dwarf reaches its maximum mass while remaining stable—a relationship known as the Chandrasekhar limit. Or, in what is known as the double-degenerate model, two white dwarves can merge their masses into one.
The increasing mass adds to the star’s density and internal pressure, and its core temperature climbs. After centuries the star reaches critical mass. Its carbon and oxygen ignite in a fusion reaction deep inside, and a thermonuclear runaway begins. For roughly a century the dwarf interior bubbles and churns as convection carries away the energy generated by carbon fusion. Hot gases rise, cool, drift back to the reaction zone, and are reheated. Eventually convection cannot carry away the heat fast enough. The burning becomes isolated and rapidly escalates. Temperature rises in a small region from hundreds of millions of degrees to ten billion degrees. A deflagration—a moving flame front of burning gases—is born.
At this point the star has reached full runaway mode and will soon explode. In the second before the final runaway, ashes rapidly rise like a giant mushroom cloud. The floating deflagration approaches the speed of sound as it rips through the surface of the star.
Simulations suggest that this breakout accelerates the subsonic burning into a supersonic detonation. The burning happens in a strong shock wave that rebounds to the unburned areas, completely incinerating the star. It then explodes violently, leaving behind rapidly expanding radioactive gases. An isotope of nickel, which decays first to cobalt and then to iron, keeps the debris hot and as bright as a medium-sized galaxy before fading away over months. The star’s life is over.
Replicating supernovas in the lab
Previous simulations in two dimensions by Woosley and Daniel Kasen from the University of California–Berkeley and Lawrence Berkeley National Laboratory (LBNL) have explained the observed asymmetrical explosions of the Type Ia.
“We found the most popular kind of supernova model—the Chandrasekhar mass model—doesn’t ignite in the dead center of the star,” said Woosley. “The explosion, once ignited off-center, stayed off-center and the supernova blew up asymmetrically, one side before the other.” This knowledge explains the differences in the appearance of supernovas when viewed from various directions and must be considered when calibrating the standard candle.
Jaguar is now running three-dimensional (3D) simulations with varying ignition-point locations, focused on the two-stage, deflagration-to-detonation sequence.
Because of the size of the computational runs, the studies are decoupled and done in three successive stages—ignition, explosion, and supernova. The team is using three codes developed for this project: MAESTRO, CASTRO, and SEDONA. John Bell and Ann Almgren at LBNL wrote MAESTRO to model the ignition and CASTRO to model the explosion. SEDONA, written by Kasen, is a hydrodynamics code that calculates the spectra and light curves of the supernovas.
The multiyear project was allocated 50 million computing hours on Jaguar in 2011 and 47 million in 2012 through the INCITE program, which is jointly managed by the US Department of Energy’s Leadership Computing Facilities at Argonne and Oak Ridge National Laboratories.
Additionally, Woosley has begun investigating another variety of Type Ia supernova—a white dwarf that accretes a thick layer of helium from a companion star. This helium-rich celestial object may detonate in a straightforward blast after an ignition in the star’s outer layer compresses its inner core.
After the simulations at ORNL blow up several 3D models of white dwarf stars and their light curves and spectra have been determined, the findings will be put into an archive available to researchers. Observers will especially analyze the data to find other, more accurate indicators of luminosity to compare with a rapidly growing library of observational data. The data will also be used to help plan future large surveys of supernovas by missions such as the Large Synoptic Survey Telescope and the Palomar Transient Factory. Said Woosley, “It is our eventual ambition to have hundreds of model supernovae sampled at all wavelengths, angles, and times available for comparison by machine intelligence with thousands of observations in order to get the very best distance measurements possible.” —by Sandra Allen McLean
Related Publications
D. Kasen, F. K. Röpke, and S. E. Woosley. 2009. “The Diversity of Type Ia Supernovae from Broken Symmetries.” Nature 460:869.
A. Nonaka, A. J. Aspden, M. Zingale, A. S. Almgren, J. B. Bell, S. E. Woosley. 2012. “High-Resolution Simulations of Convection Preceding Ignition in Type Ia Supernovae Using Adaptive Mesh Refinement.” Astrophysical Journal 745:73.
S. Blondin, D. Kasen, F. K. Röpke, R. P. Kirschner, K. S. Mandel. 2011. “Confronting 2D Delayed-Detonation Models with Light Curves and Spectra of Type Ia Supernovae.” Monthly Notices of the Royal Astronomical Society 417:1280.