The following design policies and guidelines apply to all systems within the mechanical engineering discipline. The purpose is to provide uniformity of design based on the established NIH Architectural and Engineering Design Policy and Guidelines. Systems may include heating, ventilation, and air-conditioning (HVAC), piping, insulation, and automatic controls.On this page:
F.1 Reference Design and Safety Guidelines for the HVAC Designer
F.2 Building Design Considerations
F.3 Noise and Vibration
F.4 Mechanical Equipment Location and Access
F.5 Systems Identification
F.6 Piping Systems
F.7 Insulation Systems
F.8 Testing and Balancing
F.9 Program Equipment
F.10 Motors and Drives
F.11 HVAC Systems
F.12 Fuel Supply
F.13 Steam Systems
F.14 Chilled-Water Systems
F.15 Hydronic Heating Systems
F.16 Fume Hoods and Biological Safety Cabinets
F.1 Reference Design and Safety Guidelines for the HVAC Designer
The NIH is a progressive and dynamic biomedical research institution where state-of-the-art medicine is the standard practice. To support state-of-the-art research and medical care, the facilities must also be state of the art. It is the NIH intent to build and maintain the physical plant and facilities in accordance with the latest standards. It has been the NIH experience that renovation and rehabilitation of existing facilities do not lend themselves to incorporating the “latest” standards of the industry, primarily because of outdated and inadequate mechanical systems or because the planned function is incompatible with the original criteria for the facility.
The architect and engineer (A/E) will be alerted to this type of situation and make an evaluation early in the design stage to determine the feasibility of implementing the latest standard. The A/E should document such findings, provide recommendations, and report them to the Project Officer for a decision on how to proceed.
The A/E design firm should use and comply with, as a minimum, the latest issue of the following design and safety guidelines. In addition, the A/E should use other safety guidelines received from the NIH Project Officer or as required by program. The A/E should utilize the latest versions of guidelines available at the time the project proceeds with schematic design.
The design and safety guidelines include, but are not limited to, the following:
- The International Building Code.
- The International Mechanical Code.
- The International Energy Conservation Code.
- International Code Council, Inc., and Building Officials and Code Administrators (BOCA) International, Inc.: 4051 W. Flossmoor Road, Country Club Hills, IL 60477-5795.
- American Society of Heating, Refrigerating and Air-Conditioning Engineers (ASHRAE), Inc.: 1791 Tullie Circle, NE, Atlanta, GA 30329.
- ASHRAE Handbooks and Standards.
- Industrial Ventilation: A Manual of Recommended Practice: American Conference of Governmental Industrial Hygienists, 6500 Glenway Avenue, Building D-7, Cincinnati, OH 45211.
- Occupational Safety and Health Standards, CFR 29, Part 1910:U.S. Department of Labor, Occupational Safety and Health Administration (OSHA).
- Guidelines for Research Involving Recombinant DNA Molecules: U.S. Department of Health and Human Services, U.S. Public Health Service, National Institutes of Health, Federal Register/Vol. 51, No. 88: 16957-16985, Bethesda, MD: National Institutes of Health.
- Standard 49 for Class II (Laminar Flow) Biohazard Cabinetry: National Sanitation Foundation Joint Committee on Biohazard Cabinetry, Ann Arbor, MI: National Sanitation Foundation.
- Ventilation Design Handbook on Animal Research Facilities Using Static Microisolators, Volumes I and II, September 1998, Farhad Memarzadeh, Ph.D., P.E. National Institutes of Health, Bethesda, MD: Office of Research Services.
- Methodology for Optimization of Laboratory Hood Containment, Volumes I and II, November 1996, Farhad Memarzadeh, Ph.D., P.E. National Institutes of Health, Bethesda, MD: Office of Research Services.
- Assessing the Efficacy of Ultraviolet Germicidal Irradiation (UVGI) and Ventilation in Removing Mycobacterium Tuberculosis, November 2001, Farhad Memarzadeh, Ph.D., P.E. National Institutes of Health, Bethesda, MD: Office of Research Services.
- Ventilation Design in Animal Research Facilities Using Static Microisolators, Farhad Memarzadeh, Ph.D., P.E., Gerald Riskowski, Ph.D., P.E., ASHRAE Transactions 2000, Volume 106, Part 1, 859-866.
- Investigation of Static Microisolators in Wind Tunnel Test and Validation of CFD Cage Model, Farhad Memarzadeh, Ph.D., P.E., Gerald Riskowski, Ph.D., P.E., ASHRAE Transactions 2000, Volume 106, Part 1, 867-876.
- Analysis of Air Supply Type and Exhaust Location in Laboratory Research Facilities Using CFD, Andrew Manning, Ph.D., Farhad Memarzadeh, Ph.D., P.E., Gerald Riskowski, Ph.D., P.E., ASHRAE Transactions 2000, Volume 106, Part 1, 877-883.
- Methodology for Minimizing Risk from Airborne Organisms in Hospital Isolation Rooms, Farhad Memarzadeh, Ph.D., P.E., Jane Jiang, Ph.D., ASHRAE Transactions 2000, Volume 106, Part 2, 731-747.
- Thermal Comfort, Uniformity, and Ventilation Effectiveness in Patient Rooms: Performance Assessment Using Ventilation Indices, Farhad Memarzadeh, Ph.D., P.E., Andrew Manning, Ph.D., ASHRAE Transactions 2000, Volume 106, Part 2, 748-761.
- Laboratory Safety Monograph: A Supplement to the NIH Guidelines for Recombinant DNA Research, U.S. Department of Health and Human Services, U.S. Public Health Service, National Institutes of Health, Bethesda, MD: National Institutes of Health.
- Guidelines for Laboratory Design: Health and Safety Considerations, 3rd Edition. DiBernardinis, L., and J.S. Baum, M.W. First, G.T. Gatewood, and A.K. Seth. September 2001. New York: John Wiley and Sons.
- Biosafety in Microbiological and Biomedical Laboratories: U.S. Department of Health and Human Services. Washington, DC: Public Health Service, Centers for Disease Control, and National Institutes of Health, HHS Pub. No. (NIH) 99-8395, IVth Edition, April 1999.
- NIH Guidelines for the Laboratory Use of Chemical Carcinogens: U.S. Department of Health and Human Services, Bethesda, MD: National Institutes of Health, NIH Pub. No. 81-2385.
- National Fire Codes, all volumes, National Fire Protection Association (NFPA), 1 Batterymarch Park, Quincy, MA 02269-9101.
- Guide for the Care and Use of Laboratory Animals, Institute of Laboratory Animal Resources, National Academy of Sciences. Washington, DC: National Academy Press.
- Guidelines for Design and Construction of Hospital and Health Care Facilities, American Institute of Architects Committee on Architecture for Health with assistance from the U.S. Department of Health and Human Services. American Institute of Architects Press, 1735 New York Avenue, NW, Washington, DC 20006.
- Medical Laboratory Planning and Design: College of American Pathologists, Skokie, IL.
- American Society for Healthcare Engineering, all volumes: American Hospital Association, One North Franklin, 28th Floor, Chicago, IL 60606.
top
F.2 Building Design Considerations
The project engineer should include at the completion of the schematic design phase a Basis of Design report. The report should be a bound presentation with documentation sufficiently complete to justify the complete design concept of the A/E. Detailed building design criteria, computations, schematic system diagrams, commissioning plan criteria, economic analysis, and life-cycle costing comparisons shall be included as a part of the Basis of Design report.
F.2.1 Energy Conservation: The International Energy Conservation Code should be utilized to regulate the design and construction of the exterior envelopes and selection of HVAC, service water heating, electrical distribution and illuminating systems, and equipment required for the purpose of effective use of energy, and shall govern all buildings and structures erected for human occupancy. When requirements of the energy conservation code cannot be satisfied because of program requirements, the NIH Project Officer should be notified. Refer to General Design Guidelines, Section: Sustainable Design, for energy conservation guidelines to be followed for the design of NIH buildings.
top
F.3 Noise and Vibration
These design guidelines are intended to provide general information about noise and vibration control to the A/Es charged with designing mechanical and electrical systems for the NIH. They cover situations that arise in the design process and significant items to check at design reviews. As a supplement to other sources of technical information, such as the Sound and Vibration Control section of the ASHRAE Applications Handbook and the advice of an acoustical consultant, they are intended to help the engineers achieve appropriate sound and vibration levels required by the program functions.
F.3.1 Background Noise: All rooms in all buildings, except special acoustical laboratories, are exposed to some level of audible and measurable ambient sound. It may be due to nearby street traffic but more often is governed by the building’s own mechanical and electrical systems. Ambient sound should be, and usually is, anonymous in character. This is an accepted acoustical condition to which we are almost always exposed. The ambient sound should never be so loud as to interfere with speech or telephone use in a space. Frequently, modest levels of ambient sound are needed to mask distracting extraneous sounds.
Noise is characterized by a certain spectrum indicating the sound pressure level at various frequencies. Very often, the spectrum of a noise is as important as its absolute level. Although speech and airplane takeoff may be perceived as being about the same loudness, it is much more difficult to attenuate the lower frequency noise. The level of such background sounds is commonly related to a series of noise criteria (NC) or room criteria
(RC) curves. These spectra have been developed to account for the approximate sensitivity of the human ear to high-frequency noise over low-frequency noise and also to the typical spectrum of human speech. The NC/RC value for a given spectrum is then determined by its highest point in relation to the NC curves. To determine the NC/RC value in the field, sound pressure levels should be measured with an octave-band sound-level meter.
For most spaces, recommended NC/RC levels have been established through many years of experience. In general, for areas where listening is critical and speech communication is important, the NC/RC level should be low. For areas where speech is at close distances (1.8-3.0 m), the NC/RC level may be higher. Table F.3.1 lists recommended NC levels for a variety of spaces. NC levels are based on rooms not being occupied and with all user equipment off.
Table F.3.1 Recommended NC Levels
Area |
NC Level |
Auditoriums |
20-25 |
Audiology suites, audio/speech, pathology, and phonology/cardiac |
25 |
Chapel and chapel meditation |
25 |
Private residences |
25-30 |
Conference rooms |
25-30 |
Hospital rooms |
25-35 |
Patient rooms |
35 |
Executive offices |
30-35 |
Classrooms |
30-35 |
Open-plan offices |
35-45 |
Dining rooms, offices, and lobbies |
40 |
Central sterile food service/serving |
45 |
Operating rooms |
40-45 |
Research laboratories |
40-45 |
Corridors and support areas |
45 |
Kitchen, lockers, warehouse, and shops |
50 |
Research animal housing areas |
see note |
Note. When evaluating the noise levels in research animal housing areas, it is necessary to consider both the people and the animals in these spaces. For reasonable speech communication in these spaces, a maximum noise level of NC-45 should be maintained. The acoustical consultant should determine specific requirements for animal research areas with the Project Officer and research staff.
The above NC values may be increased for unitary or user equipment installed within occupied spaces as approved by the Project Officer. The sound levels apply to these spaces in most common situations. If the users of the space are hearing impaired, then the tolerance for high background noise levels is greatly reduced. For situations involving audiological testing, there are very specific requirements. In either of the latter two cases, an acoustical consultant should be involved in the design at an early stage. Systems must be engineered and the use of sound attenuation provided as required to achieve specified sound levels.
Both the project engineer and the Government should be aware of the costs and benefits related to the choice of noise criteria or room criteria curves. Studies have shown RC curves to have a more desirable spectrum for background noise. However, RC curves may require more noise reduction in lower octave bands than would be required for an NC curve. This noise reduction may entail significant costs in equipment and operations. For spaces at about NC-30 or NC-40, the duct silencers will be 1.5 to 2.0 times longer and pressure drop will increase 10 to 20 percent. If architectural constructions are used for noise control, they will also be more massive and elaborate. In general, NC curves provide a more reasonable fit between costs and benefits and should be utilized for NIH buildings.
F.3.2 Scientific Equipment Noise: The design team should be aware that many noise sources in health care and research facilities are not related to the building mechanical system. NIH buildings are often equipped with refrigerators, centrifuges, and other scientific equipment that contribute significantly to the ambient noise level. In some cases, this equipment will be located in service corridors and adjacent to occupied spaces.
Most of this equipment operates intermittently and is often under the control of the user. Since the types of equipment vary greatly, it is not possible to prescribe a single means of noise control. We recommend that equipment that produces significant amounts of noise be considered during the design stage. Any equipment that either produces noise levels in excess of 50 dB (A) at a distance of 914 mm, or is simply known by the laboratory users to produce objectionable noises, should be considered a significant noise source.
For adequate speech intelligibility at a distance of 1.8 m, with normal voice effort, the background noise level should not exceed 50 dB (A) or NC-45. In addition, the background noise spectrum should not interfere with speech intelligibility. Since the bulk of speech intelligibility is in the 500 to 4 000 Hz octave bands, sound levels should be lower in that area. A goal of NC-45 is equal to 53 dB (A) and has been designed to account for the
frequency distribution of speech intelligibility. NC-45 should be used as a maximum design goal for occupied research areas in NIH buildings.
As noted previously, refrigerators and other scientific equipment are frequently removed from occupied areas and placed in corridors. In many cases, this alone can cause fairly high noise levels. Because of this, noise levels of NC-45 in corridors and support areas are recommended.
F.3.3 Background Noise for Open Offices: Most office workers have difficulty concentrating when distracted by conversations and intruding noises. In open-plan offices, voices and the sounds of other activities are easily transmitted between workstations because there are no full-height partitions or barriers. Even with the most effective acoustical treatment on the partial-height partitions and ceilings, the intruding sounds will be clearly audible unless they are masked by other sounds. For this reason, providing such masking sound is essential in the design of any open-plan office where speech privacy or the ability to concentrate is important. Even in buildings with conventional enclosed offices, full-height partitions may not provide adequate acoustical isolation for confidential speech privacy if the background sound levels are too low. Installation of a sound-masking system can be the least expensive, simplest way to achieve satisfactory privacy.
For satisfactory performance, a sound-masking system must provide an even blanket of sound throughout the office. The masking sound must be free of annoying spatial or temporal differences in loudness and must provide specific levels of sound at specific frequencies. At the same time, it must be unobtrusive in overall level and character so that it is not, in itself, an annoyance to the office occupants. Elevated background noise levels
can, however, cause problems for hearing-impaired employees. If it is known that one or more hearing-impaired employees will be working in an area, then the designer should endeavor to provide a space with low background noise levels and a significant amount of acoustical absorption. If a sound-masking system is provided, the use of adjustable levels in zones can be beneficial.
F.3.4 Design Guidelines: The evaluation of mechanical system noise should take place in the early design phase of a project. This evaluation is as important as thermal load calculations. System noise calculations are the responsibility of the project engineers designing the system, unless an acoustical consultant is employed. It is not recommended that a project engineer without experience in acoustical matters attempt an acoustical analysis of a major project. It is the experience of the NIH that about two-thirds of these analyses are wrong in very elementary ways, and the monetary consequences to correct acoustical deficiencies can be substantial.
F.3.5 Noise Control: For most large buildings, there will be three types of mechanical rooms: central mechanical rooms, interstitial spaces, and individual floor mechanical rooms. To begin an analysis of the requirements for sound attenuation and vibration isolation of the mechanical room, two items must be identified. The first is requirements of adjacent rooms, both in plan and in section. The second is the type and size of equipment in the mechanical room. The selections need only to be general at this point. Reasonable sound-level estimates can be made without specific manufacturer’s model numbers for standard equipment.
The ASHRAE Applications Handbook Sound and Vibration Control section allows the project engineer to make general estimates of equipment noise levels. For significant equipment, manufacturers should be asked to provide laboratory-generated sound power levels. These should then be incorporated into the equipment requirements of the project specifications. In many cases, it may be possible to minimize expensive noise control measures if quieter equipment can be selected.
At this point, it is possible to estimate the noise reduction requirements for the mechanical room. If a mechanical room is to be located below or above a noise-sensitive space, this should be identified early in the design. If the floor slab above or below mechanical equipment is not sufficiently massive to provide adequate noise isolation, then it may be difficult to modify it after the structural system has been sized and set. At this point, two solutions are often used. One is a floating floor and the other is a resiliently suspended gypsum board ceiling in the mechanical room or in the noise-sensitive space below. The first is very expensive, and the second is very difficult to install properly in the equipment room below because of pipes, conduit, and equipment. Spaces underneath mechanical rooms face similar problems, but it is generally easier to install a suspended gypsum ceiling in a conference room or office than in a mechanical room.
If possible, the mechanical room should be located away from noise-sensitive spaces. Buffer spaces such as corridors, toilets, elevator shafts, electric closets, and other service spaces may eliminate the need to build special noise-isolating constructions such as floated floors or double-layer wall constructions. In all cases, central mechanical rooms in occupied buildings should have heavy walls of masonry or poured concrete. All penetrations of walls, floors, and ceilings by ducts, pipes, conduit, and so on should be resiliently sealed airtight. Particular attention should be paid to doors, as these often represent the “weak link” in sound isolation. A good-quality gasket system to minimize the maintenance problems may be used where required to seal air leaks. Mechanical and electrical equipment spaces located within NIH buildings should preferably be designed without additional sound treatments. When a mechanical room is near noise-sensitive areas, it shall have a soundabsorbing treatment installed on the walls and ceiling. At least 30 percent of the available wall surfaces and 50 percent of the ceiling surface should be covered with a soundabsorbing treatment. The preferred material is a glass fiberboard with a density in the range of 24 to 64 kg/cm. Other sound-absorbing materials can be used, except cellular plastic materials, and these should provide a minimum noise reduction coefficient (NRC) of 0.65 for a 25 mm thickness and a minimum NRC of 0.80 for 50 mm and 75 mm thicknesses as determined by American Society for Testing and Materials (ASTM) C423. They should also provide a minimum flame-spread rating of 25 and a minimum smoke-developed rating of 50 as determined by ASTM E84.
The minimum thickness of the sound-absorbing glass fiber material used in equipment spaces should be as follows:
Table F.3.5.a Minimum Thickness of Sound-Absorbing Treatment
Space Contents |
Minimum Thickness of Sound- Absorbing Treatment (mm) |
Boilers and emergency generators |
75 |
Chillers and fans |
50 |
Pumps, compressors, transformers, elevator, MG sets, and switchgear |
25 |
Consideration should be given to the application of enclosures or jackets over generators to provide additional attenuation for equipment operators within the space. Sound-absorbing treatment also reduces the noise levels in mechanical equipment and other high noise-level spaces and helps reduce the possibility of hearing damage to maintenance personnel. Tabulated below are the maximum allowable noise exposure limitations for hearing conversation of individuals in high noise-level areas, as defined by the Occupational Safety and Health Administration (OSHA).
Table F.3.5.b OSHA Sound Level Limits
Exposure Duration (h/d) |
Sound Level OSHA Limits (dB A) |
8 |
90 |
6 |
92 |
4 |
95 |
3 |
97 |
2 |
100 |
1½ |
102 |
1 |
105 |
½ |
110 |
¼ and less |
115 |
F.3.5.1 Airborne Noise Control: In designing a building HVAC system, it is most common to size ductwork on an equal-friction basis and consider velocities indirectly, as they relate to the volume flow and pressure drop in the ducts. For purposes of noise control, it is often necessary to consider duct velocity for its own ability to generate noise in a system. (Velocities in airflow generate turbulence and therefore noise.) The amount of noise generated is proportional to 50 x log (velocity). Because of the uncertainties involved in calculating exact velocities through elbows, dampers, and other fittings during the design process, it is often best to use general guidelines. The path of noise to any potential receiver should be examined. In most cases, the dominant path for noise is through the duct to a room outlet. In more severe cases, noise from turbulence may “break out” of the duct and enter a space directly. The final general area to consider is the acceptable noise level at the receiver location. Duct velocities serving auditoriums must be considerably less than those serving research laboratories.
The selection of quieter, initially more expensive equipment is generally more economical than a less expensive type, which requires considerably more noise and vibration control. Measured sound-power ratings should be supplied by the manufacturer and should be a factor in the selection of each major piece of mechanical equipment. Low- or medium-air velocity systems should be used. Low- velocity distribution requires less energy to move the air and also greatly reduces the generation and regeneration of noise produced by high velocities.
Table F.3.5.1 lists recommended velocities for ductwork serving spaces with a given NC rating. When measuring distance from the air terminal, it is important to measure from each terminal, not just the last one. In this case, the “terminal” is a bit different from what is used for static pressure calculations. These velocities may be increased if all paths to the receiver from the turbulence in the duct are considered. In general, this means installing a silencer along the duct. To prevent breakout noise, a duct enclosure or architectural construction must also be used.
Table F.3.5.1 Recommended Maximum Air Velocity in Duct System
Location |
Maximum Air Velocity (m/s) |
NC-25 |
NC-30 |
NC-35 |
NC-40 |
NC-45 |
|
Supply |
Return |
Supply |
Return |
Supply |
Return |
Supply |
Return |
Supply |
Return |
Air velocity through net-free area of terminal device, 13 mm minimum slot width |
1.78 |
2.13 |
2.16 |
2.59 |
2.54 |
3.05 |
3.05 |
3.66 |
3.66 |
4.37 |
3 m of duct before opening |
2.13 |
2.49 |
2.59 |
3.05 |
3.05 |
3.56 |
3.66 |
4.27 |
4.37 |
5.08 |
Next 3 m |
2.84 |
3.20 |
3.45 |
3.89 |
4.06 |
4.59 |
4.88 |
5.49 |
5.84 |
6.60 |
Next 3 m |
3.56 |
4.06 |
4.32 |
4.93 |
5.08 |
5.28 |
6.10 |
6.35 |
7.32 |
7.62 |
Next 3 m |
4.57 |
4.98 |
5.49 |
6.00 |
6.50 |
7.11 |
7.78 |
8.53 |
9.35 |
10.23 |
Next 3 m |
5.19 |
6.40 |
6.91 |
7.82 |
8.13 |
9.14 |
9.75 |
10.97 |
11.68 |
13.21 |
Next 3 m |
7.11 |
7.82 |
8.63 |
9.50 |
10.16 |
11.18 |
12.19 |
13.41 |
15.24 |
16.10 |
Note: Velocities for exhaust systems should refer to recommended return velocities unless higher velocities are required by Industrial Ventilation Standards.
F.3.5.2 Rooftop Equipment: For some buildings, packaged rooftop or commercial-grade unitary equipment may be used rather than having equipment located in central or individual mechanical rooms. Special care should be taken in the location, selection, and design of this type of equipment. The roof structure should be sufficiently stiff that it does not vibrate with the equipment. Most commonly used vibration isolation selection tables assume a reasonably stiff supporting structure. In the case of many lightweight roofs, that assumption is neither safe nor accurate. From an acoustical viewpoint, the preferred mounting arrangement is to place the unit above the roof by 610 mm or 915 mm on supplemental steel framing. Equipment manufacturers’ internal vibration isolation furnished as standard or optional equipment may not be adequate for controlling the transmission of noise and vibration. The required deflection should be maintained for either internal or external isolation. However, manufactured, spring-isolated roof curbs are available with integral isolation. These units can provide spring deflections ranging from 6 to 75 mm and can be used as an acceptable option. Curb isolation may be adequate, but proper isolator selection is important to compensate for each building construction condition. In addition to structural vibrations, the noise radiated from the unit casing and the supply and return ductwork must be considered. In most of these cases, there is a potential noise problem that would almost always be worst directly under the unit. In addition to these unique problems, normal duct-borne fan noise should also be considered. All of this is not a reason to eliminate the use of rooftop equipment, but it is necessary to review these points to properly evaluate all these potential problems. It is recommended that housed-type vibration isolation mounts not be used.
F.3.5.3 Noise Outside Equipment Rooms: Many noise problems with mechanical systems are associated with that part of the building just outside the equipment room. This type of noise is generated in two ways. The most common is noise generated by the fan that is propagated within the ducts to outlets. The second type is noise generated by air turbulence at fittings, vanes, and dampers. High-pressure, high-velocity systems will often have significant quantities of both types of noise and vibration. The noise generated by ducted systems will typically enter spaces in three ways. It may pass through the duct walls and into noise-sensitive spaces. It may travel within the ductwork and enter a space through supply or return grilles. Finally, vibrations in the duct may be transmitted into other surfaces or utility systems to either create noise or become perceived vibrations. This final type will be covered in the Vibration Isolation paragraph.
Noise that passes through duct walls is usually referred to as “breakout” or “breakin” noise. This noise may be either fan noise or velocity-generated fitting noise. This is often a problem closest to the fan. At this point, the ducts are large and therefore not very stiff. Near the mechanical room, noise from the fan has not been attenuated by long runs of ducts. This usually causes a low-frequency rumble in the vicinity of main ducts, especially if the duct is directly above a lay-in acoustical tile ceiling. Exposed duct, in itself, does not create a noise problem. In the case of exposed ducts, breakout noise would not be attenuated by a ceiling. However, the noise reduction provided by a lay-in ceiling is negligible at low frequencies. Calculations based on the ASHRAE Applications Handbook Sound and Vibration Control section should be performed to determine the likelihood of a problem. Should there be a problem, several methods can reduce the potential noise level. First, the duct may be rerouted over a noncritical area. Second, round duct or multiple round ducts may be used in lieu of rectangular duct if adequate space is available, since round duct is stiffer. Third, the duct may be externally wrapped or encased. The final two methods are difficult to do well.
Duct wrappings may encounter sufficient numbers of obstructions and penetrations to render them ineffective. Accessing valves and duct-mounted equipment becomes difficult. While wrapping can be effective, it should be employed only when absolutely necessary.
F.3.5.4 Duct-Borne Noise: The passage of noise from the fan along the inside of the duct and into a space is one of the most common noise problems associated with mechanical systems. The engineering procedures to deal with this problem are also well documented in the ASHRAE Applications Handbook Sound and Vibration Control section. It should be pointed out that this method can also be used to calculate the propagation of noise generated at fittings and dampers away from the fan. This is particularly relevant in the design of laboratory exhaust systems, where the velocities and pressures in the systems are often quite high. In these circumstances, high levels of noise may be generated at fittings and volume-regulating dampers. For laboratory systems in particular, this noise should be included in the acoustical analysis of the system. Within the duct system, several items provide some attenuation of noise. These are branch takeoffs, open-end reflections, fittings, and duct silencers. These are all discussed in detail in the ASHRAE Applications Handbook, so only some minor points will be discussed here. Branch takeoffs provide a division of sound energy proportional to the decibel ratio of the areas involved. For example, assume the room in question is served by a 508 x 254 mm (129 032 mm2) branch from a 1 219 x 1 219 mm (1 485 961 mm2) trunk. The attenuation provided is 10 x log (200/200 + 2,304) or 11 dB. This credit should be taken only where one of the branches does not enter the room in question. Where a fan serves many rooms, this can be a substantial help. Duct lining is one of the most efficient noise control measures available, but lining is not approved for use in NIH buildings.
Manufactured duct silencers are another commonly used means of noise control. These are commercially manufactured sound absorbers. They consist of a section of sheet metal with perforated interior skin and sound-absorbing in-fill and are available in many constructions, sizes, and shapes. In general, these factors can be matched to the requirements of the system under design. In the design of hospital, animal, and laboratory systems, it may not be appropriate to allow standard perforated, fiberglass-packed, galvanized silencers. Alternately, a requirement for high-grade stainless steel, packless washable silencers may be necessary. Silencer manufacturers can also provide thin plastic bags for the fill. They also provide a thin mesh screen between the bagged fill and the perforated metal baffles to ensure minimum degradation of acoustical performance. Insertion loss and spectrum level are also important characteristics when selecting duct silencers. Generally, the 125, 250, and 500 Hz octave-band center frequencies are most critical. Duct-borne sound-level calculations will provide the required insertion loss for a silencer. Sample calculations provided by some manufacturers will often show a very close match between the octaveband insertion loss requirements and the performance of the silencer that is chosen. This does not usually happen in real-world situations. The insertion loss requirement for the silencer will usually be dominated by one or two low-frequency octave-band center frequencies depending on fan type, blade passage frequency, and blade configuration, which affect the fan sound-power level. The other factors in silencer performance that should be considered are size and pressure drop. It is usually possible to meet the insertion loss requirements with several different-sized silencers. That choice is usually between a long, low-pressure drop silencer and a shorter, high-pressure drop silencer. At this point, the engineer must make a choice between system operating cost and first cost. Once a silencer is selected, it must be incorporated into the duct layout. The silencer should be located so that smooth airflow is maintained into and out of the silencer. Poor design in these areas can cause the actual pressure drop to be much more than that listed by the manufacturer and can also degrade acoustical performance. Proper specifications should require ratings in dynamic insertion loss (DIL), i.e., with air flowing through the silencer. All supply and return exhaust air (research laboratory and animal research facilities only) systems shall use packless type silencers. Silencers such as IAC type HS may be used for all supply boxes if they are clean flow boxes with Tedlar or other coverings conforming to NFPA 90 standard over perforated metal cover liner with an erosion-proof surface meeting ASTM C1071-91 test. The liner must have passed and shown no observed growth for the test for mold growth and humidity using UL 181, fungi resistance ASTM C1071, and ASTM G21 tests and bacteria growth using ASTM G22 test.
It is important in locating the silencer to keep in mind that any noise generated downstream or upstream in the case of exhaust systems from the silencer will not be attenuated. For a laboratory system, it is important to remember that constant-volume-regulating dampers will usually generate a substantial amount of noise, especially if there is a substantial pressure drop (more than about 25 mm) across the regulating damper. If the silencers are placed near the fan, then noise generated by these dampers will enter the laboratory unattenuated by the silencers. For spaces with critical listening requirements, such as auditoriums and large conference rooms, similar problems can be created by excessive velocities at supply
or return terminal devices. Since the amount of noise is velocity related, it is advisable to elect terminal devices with more free area for critical spaces.
F.3.6 Equipment Noise: In some cases, the engineer may be concerned with noise from relatively small pieces of equipment, particularly if they are located in an occupied space rather than in a remote mechanical room. These include “active” devices and “passive” devices. Active devices are most often items such as fan coil units, heat pumps, or air terminal units. Passive devices are most often diffusers, air-monitoring devices, grilles, and louvers. For the active devices, most manufacturers can provide octave-band sound-power levels. For passive devices, manufacturers’ ratings may also be provided. In general, these can be used if some attention is paid to the quantity of diffusers in a room. Hoods with velocities around 0.76 m/s will almost never be a direct cause of noise. System noise may come out of the hood, though. The same is true of louvers. Noise may often be heard coming out of these devices, but they are not often the actual cause of the noise.
F.3.7 Vibration Isolation: Structure-borne sound is produced by a noise source, such as a piece of vibrating machinery, that transmits energy directly into and through the structure, often to remote locations in a building, and is reradiated by wall and floor construction as airborne noise. All vibrating equipment in facilities must be resiliently mounted.
The purpose of vibration isolation is to reduce the vibrational energy produced by rotating equipment so that it is not passed into the structure and into larger “sounding boards” where it can be translated into audible noise. In the case of some sensitive scientific equipment, structural vibrations may be harmful to its operation. This is true even in some cases where the frequency and level of the vibration are so low that they cannot be felt but measured only with sophisticated instrumentation. When a project involves the use of vibrationsensitive equipment, such as electron microscopes, a vibration specialist should always be consulted. The ASHRAE Applications Handbook Sound and Vibration Control section contains guidelines and a table of vibration isolation selections for most common situations.
Space requirements for the isolation springs and equipment bases should be included in the equipment layout. At least 50 mm of horizontal and vertical clearance should be provided between all isolated equipment and the building structure. More space is usually preferred for proper access for installation and adjustment. If equipment can be located in an area that is as stiff as possible, then vibration isolation requirements will be minimized. Equipment that is located on grade is preferred; if that is not possible, then areas above stiff major beams are the second-best location. For standard mechanical equipment, the location is most important for large equipment with a slow rotational speed. For very lightweight mounting surfaces, particularly roof decks, it may be necessary to provide separate framing for the mechanical equipment.
Housekeeping pads are usually provided under all floor-supported equipment. The pads should be connected to the slab with steel dowels. The pad area may be sized to extend beyond the resilient mounts of isolated equipment. These pads are intended to provide local mass and stiffness below mechanical equipment and to keep resilient mounts off the floor, where they may be easily blocked by debris under the spring or equipment bases.
Four basic types of vibration isolators or resilient mounts are resilient pads, elastomeric mounts, steel springs, and pneumatic mounts. Each type has advantages over the others depending on the degree of isolation required, loadings, flexibility of the supporting structure, and driving frequency.
- Resilient Pad Mounts. Resilient isolators are the easiest and most commonly used material. Resilient pad mounts are available in a variety of materials such as ribbed or waffled neoprene and rubber, precompressed, load-bearing glass fiber, felt foam, and cork. For maximum life and durability, pads of rubber, neoprene, or glass fiber should be used. Care shall be taken, however, in the selection of the proper material type, density, thickness, and size to ensure that the appropriate loading of the material is achieved. Overloading a resilient pad material causes increased stiffness of the pad and thereby significantly reduces its isolating effectiveness.
- Elastomeric Mounts. General-purpose elastomeric mounts typically consist of a resilient material such as neoprene, which can be easily molded into special shapes. These mounts shall be bonded to metal plates and support members of the equipment.
- Steel Spring Isolators. The most effective vibration isolating devices available are steel-spring mounts, particularly where large pieces of equipment are involved.
- Pneumatic Mounts. Where low-natural-frequency mounts are required, pneumatic vibration isolators should be used. In this type of mount, an elastomer is combined with air to form a rubber/air spring. Pneumatic mounts provide both support and resilience for the equipment mounted on them. By proper sizing and distribution, a very stable, lowprofile and low-natural-frequency isolator mount can be obtained with built-in shock overload protection and built-in damping and, in certain cases, without the need for external lateral stability provisions.
F.3.8 Equipment Installation: Mechanical equipment with a high power-to-weight ratio should first be mounted on a concrete inertia base approximately 1 to 2 times the weight of the equipment, plus system fluids, if any. The inertia base and equipment should be resiliently isolated on freestanding, unhoused, stable steel springs and noise isolation pads. Typical pieces of equipment that require concrete inertia bases include fans and chillers over 18.6 kW and pumps and compressors over 3.7 kW. Fan equipment with motors smaller than 18.6 kW should be mounted on rigid structural-steel frames and the entire assembly mounted on vibration isolators plus noise isolation pads. When the building structural system cannot accommodate the added weight of concrete inertia bases, very high efficiency isolators such as pneumatic mounts should be used to isolate the equipment mounted on rigid steel frames.
Restraint for lateral and vertical seismic loadings should be achieved through the use of resilient snubbers, which are mounted outboard of the inertia base on the housekeeping pad. The snubbers shall consist of steel angles or brackets bolted to the structure with a layer of resilient material between the inertia base and steel angle. The steel angles and bolts should be sized by the structural engineer to accommodate the applicable G-force loadings (either static or dynamic) based on the design parameters of each project. Several vibration isolation manufacturers provide isolators that have integral seismic restraint elements built in. However, since inspection of the interior of the units is difficult, they are susceptible to flanking of vibrational energy due to metal-to-metal contact through misalignment.
F.3.9 Steel Spring Isolator Specifications: The most effective vibration isolation system for mechanical equipment involves mounting the equipment plus inertia base or steel frame on freestanding, unhoused, stable-steel springs, with additional travel between solid (fully compressed) height and design height equal to 50 percent of the static deflection of the spring. Housed-spring units with multiple, small-diameter coils or units with rubber or neoprene cups must not be used. The horizontal stiffness of the spring isolators shall be specified to be between 0.9 and 1.2 times the vertical stiffness, and the outside diameter of the springs shall be between 0.85 and 1.25 times the operating height of the spring. Each spring should be equipped with a resilient noise isolation pad between the structure and spring foot. The noise isolation pad shall be precompressed, molded, neoprene-jacketed, load-bearing glass fiber or multiple layers of ribbed or waffled neoprene. For mechanical equipment located on grade, the noise isolation pad shall be a minimum of 13 mm thick. At all locations above the grade level, the noise isolation pad should be at least 25 mm thick.
F.3.10 Static Deflections: The static deflections required for vibration isolators are determined by the speed and horsepower of the equipment mounted on them, as well as by the location of the equipment within the building. For this reason, it is best to locate as much of the vibrating equipment at grade level as is practicable. All mechanical equipment above grade level should be located as close as possible to or over a column, load-bearing wall, or other stiff structural member. At above-grade locations, the minimum static deflection of any steel spring used to vibration-isolate a piece of equipment shall be 25 mm. Fractional horsepower equipment should be mounted on rubber-in-shear or glass fiber isolators providing at least 13 mm static deflection.
F.3.11 Flanking Transmission: Flanking transmission of vibrational energy from mechanical equipment should be minimized. All connections to vibrating equipment shall be through flexible connectors, conduits, piping, or hose. Resilient ceiling hangers or floormounted resilient supports should support all piping in mechanical equipment spaces connected to vibrating equipment. Penetrations through equipment room walls and ceilings should be oversized, packed with a resilient material such as glass fiber or mineral fiber, caulked airtight, and covered with escutcheon plates where required for fire ratings. Piping should be supported on both sides of the penetrations and should not rest on the structure.
F.3.12 Piping Systems: One of the most common acoustical problems found in buildings is noise generated by the piping systems. Because of its easily identifiable nature, piping noise is one of the most disturbing and offensive types of noises encountered in buildings even though the levels are seldom excessively high. Most of the noise from piping systems is structure-borne, being transmitted along the piping throughout the building where the noise is reradiated as airborne noise.
Piping runs should be resiliently isolated from the surrounding structure, particularly when the piping runs are adjacent to acoustically sensitive areas such as conference rooms. Isolating materials should consist of rubber, neoprene, or spring mounts and felt- or glass fiber-lined sheet metal straps or clamps. At all wall and floor penetration and anchorage points, water piping runs should be free from the structure and the opening packed with a resilient insulation material and fully caulked. Pipes larger than 50 mm in diameter should be suspended from the structure on neoprene-in-shear hangers or floor-mounted on resilient supports. Riser piping near critical areas shall be kept free of the structure, and vertical alignment should be achieved through the use of resilient guides rather than solid anchorage to the structure. Flexible pipe connectors should be used to connect the supply and drain pipes to vibrating units such as garbage disposals, pot and pan washers, and dishwashers.
High-pressure steam and water systems are inherently noisy because of turbulence in the fluid flow. To prevent the generation of excessive flow noise cause by turbulent flow in piping systems adjacent to sensitive areas, fluid pressure should be in the range of 276 to 345 kPa. In larger facilities where high-pressure main supply lines are required, pressure regulators should be used in the supply branches at each floor to maintain the fluid pressure within the above limits. High-velocity flow in the piping system also produces turbulent flow and high noise levels. In piping runs adjacent to acoustically critical areas, such as conference rooms and patient rooms, the maximum flow velocities should not be exceeded.
The use of short air-filled branch pipes or stubs to control water hammer is not effective since the entrapped air in the stubs gradually dissolves into the water. The most efficient means of preventing water hammer is to install one of the mechanical devices manufactured for this purpose, which employs a gas-filled stainless steel bellows to absorb the shock of the hydraulic waves by mechanical compression of the bellows. These devices are available in a variety of sizes to accommodate most fixture sizes used in buildings. Another method for preventing water hammer in piping systems is to install spring-actuated or relief valves that prevent the instantaneous closure of the valve.
Steam pressure-reducing stations and other major pressure control devices can generate significant noise within mechanical rooms. Design documents should require valve manufacturers to meet a specified noise criterion with the possible use of noise suppressors. Steam pressure-reducing valves should be selected for reduced noise generation to meet design criteria. Noise suppressors should be installed when required. Acoustical attenuation adjacent to the reducing station should also be considered.
Electrical conduit connections to all isolated equipment should be made so they do not short-circuit the resilient connections. Conduits less than 25 mm in diameter should be made using flexible conduit sections forming a grossly slack connection. Larger sized connections should be made with manufactured flexible fittings.
Cooling towers on top of buildings should be placed above the roof on an independently supported steel framework. Cooling towers with large, slow propeller fans require vibration isolators with much larger, higher deflection springs than comparably sized towers with centrifugal fans. For multiple- or variable-speed equipment, the isolator critical frequency should be one-half of the slowest equipment frequency (60 rad/s). For example, a cooling tower may have a maximum speed of 12 rad/s and a minimum speed of 4 rad/s, or 40 Hz. The isolator critical frequency for that cooling tower should be less than 20 Hz.
F.3.13 Noise Control for Electrical Equipment:
- Elevators. Both hydraulic and traction elevators may be the cause of disturbing noise and vibration problems and should be evaluated during design. Hydraulic elevators should have the motor/tank/pump assemblies mounted on neoprene isolators that achieve at least 9 mm deflection. Hydraulic piping should be resiliently isolated from the building. Neoprene pad isolators should be used at pipe sleeves, pipe supports, and pipe hangers. For traction elevators, the motor/winch lifting assemblies and motor/generator sets should be isolated from the structure with constrained neoprene isolators that achieve a minimum deflection of 9 mm. Electrical connections to the isolated equipment should not short-circuit the isolation and should employ flexible conduits or fittings previously noted.
- Electric Transformers and Dimmer Banks. Transformers and dimmer banks may be sources for both noise and vibrations. Large utility distribution transformers may be a noise problem in the surrounding community because of the pure tone noise or “hum” associated with them. Smaller distribution transformers inside a building should be isolated from noise-sensitive spaces. Neoprene pads or hangers should be used to attenuate structure-borne vibrations.
- Variable-Speed Drives (VSDs). Three basic types of variable-frequency drives can be used with HVAC equipment:
- Current-source inverter type
- Voltage-source inverter type
- Pulse-width modulation (PWM) typ
The current-source type is usually the quietest. With voltage-source inverter types, generally the driver units themselves represent the noisiest source. PWM types generally make the motors on the equipment served most noisy while the drive units themselves may be very quiet. For the PWM type, drive units and motors should be compatibly matched.
F.3.14 Community Noise: During design, it is important to realize that noise created by the mechanical systems propagates outside the building, as well as inside. When the site is chosen, the location of nearby noise-sensitive neighbors should be considered. Most often these are residences, but churches, hotels, schools, and dormitories should not be neglected. There may be noise codes that apply and provide specific criteria that may not be exceeded. However, it may be desirable to use a “good-neighbor” policy and keep the noise level at or below the existing ambient condition. That level may be quite low at night, so some judgment must be used in establishing what will be considered satisfactory levels.
Several types of equipment may cause noise problems outside a building, as well as inside. The most common are emergency generators, cooling towers, roof fans, rooftop condensing units, and so on, which, if located outside, can be a problem if they are numerous or large enough. An area that is often overlooked is the exterior connection of a laboratory’s supply and exhaust fans. These fans are usually quite noisy, and the connections to the outside are generally quite short. It is important to identify significant sources early in design. These noises are most commonly treated with duct silencers or acoustical barriers.
top
F.4 Mechanical Equipment Location and Access
The project engineer should ensure that all mechanical equipment room layouts are designed to facilitate easy, quick, and safe maintenance access and replacement of system components and housekeeping. Equipment room layouts should be designed using the largest physical dimensions possible for all specified equipment. All manufacturer’s specified items should fit within the allocated space. When spacial restrictions and weight restrictions exist in equipment areas, maximum equipment dimensions and weights should be indicated on the contract documents. The NIH requires a high level of reliability from its equipment and rapid repairs when it is out of service because of operational failure. Installation of the equipment on the rooftops exposed to weather compromises the long-term reliability and can make repairs of the equipment dependent on the weather. Where possible, all equipment should be installed in mechanical rooms of adequate size to service the equipment.
Placement of equipment outside mechanical rooms must permit access from non-restricted, uncontaminated areas. Systems must be accessible for maintenance 24 hours per day, 7 days per week. Equipment requiring frequent service should not be installed in occupied rooms or above ceilings in working areas unless it is unavoidable because of space configuration, and efforts should be made to locate it in the traffic areas of the occupied space.
System plenums and casings should be designed to permit maintenance, cleaning, and replacement of all system components without disassembly of the casing. Fan replacements may be accommodated through removable casing sections. Where possible, motors, drives, lubrication devices, valves, traps, and so on should be located exterior to the plenums and casings for ease of maintenance. In no case shall motors and drives or other components requiring regular service be located within an exhaust airstream.
Easy, quick, and safe access to building utilities such as piping, valves, electrical switches, and circuit breaker panels should be provided. All valves and switches should be properly identified in accordance with the governing codes and standards. Operation and maintenance (O&M) manuals for all mechanical supplied equipment on the project are required and should be called for in the specifications. A meeting shall be specified to turn over the equipment inventory and O&M manuals to the Office of Research Facilities (ORF).
Systems should be designed in accordance with the following principles:
- Systems should be selected with minimal mechanical components requiring service and maintenance.
- System components requiring frequent service and maintenance should be located in equipment rooms or service areas and not above suspended ceilings or in occupied spaces.
- Clear and safe access should be provided for servicing, removing, and replacing equipment.
- Sufficient instrumentation should be specified for measuring, indicating, monitoring, and operating at part load as well as full load.
- Equipment should be selected for long-term durability, reliability, maintainability, and serviceability.
- Equipment should not be located in confined, or with an access through, secured spaces.
- Main service isolation valves should not be located close to the mechanical room entrance so that mains may be secured safely in the event of a system failure.
- The building design should define installation zones for piping, ductwork, conduits, cable trays, and lighting so that access to all serviceable components is clearly defined and shown on the zoning diagrams as part of the construction documents.
- All environmental room air-conditioning components must be located to accommodate service from outside the plan area of the room. Temperature and humidity sensors may be located within the rooms. Condensing units must not be located directly above the room.
top
F.5 Systems Identification
A complete identification system shall be provided for all mechanical and electrical systems/equipment that conforms with the requirements published in ANSI Standard 13.1. In existing buildings, coordinate with the existing system of identifications that may be in compliance with NFPA and NIH Specification 15011. Equipment and valves identification and numbering shall be coordinated with the ORF.
All control devices, i.e., panels, switches, starters, pushbutton stations, relays, temperature controls, and so on, should be clearly identified as to their function and the equipment controlled. All equipment such as pumps, fans, heaters, and so on should be marked to clearly identify the equipment and space or duty they serve. Equipment shall be identified using engraved, laminated black-and-white phenolic legend plates. Letters should be white on surrounding black at least 19 mm high.
Piping shall be identified with colored, prerolled, semi-rigid plastic labels set around pipes with a field-installed high-strength cement compound applied along their longitudinal edge. Labels shall be placed around the piping or insulation every 9 m and with one label on each pipe in rooms smaller than 4.5 m and on each side of penetrated wall/floor. A label shall be placed at every major valve at least 1.8 m from exit or entrance to an item of equipment and at each story traversed by the piping system. Exposed piping in mechanical rooms shall have full-color coding. Fire protection piping shall have full-color coding in all locations.
Labels shall have at least 19 mm-high black letters for pipes 25 mm and larger, and 13 mm letters for smaller pipes. All labels shall have flow arrows. Color-coding and stencil designations shall be in accordance with ANSI Standard 13.1. Where items requiring routine service are concealed above ceilings or behind access doors, a suitable and visible label should be attached to the surface to identify the location of such items. In no case shall piping be identified with generic terms, i.e., “cold water,” “hot water,” and so on. Instead, identification should be system specific, i.e., “potable or domestic cold water,” “Industrial or Laboratory Cold Water,” “Plant Air,” and so on. Each lab water outlet should be provided with a laminated identification sign that reads “Laboratory Water—Do Not Drink.” Similar signage should be provided for use at ice machines in laboratories and water faucets on nonpotable water systems.
All valves shall be provided with colored plastic, brass, or aluminum valve tags with stamped-in numbers. Tags shall be secured to the valve with a metal chain. Stop valves on individual fixtures or equipment where their function is obvious, or where the fixture or equipment is immediately adjacent, need not be so equipped. Care should be exercised in scheduling and selecting valve numbers. The number sequence should be specific and continuous with individual piping services; that is, domestic water system valves are always identified as 1.1, 1.2, 1.3, and so on, and other distinctly different piping systems should have another number series. Schematic drawings of each floor should show the approximate locations, identity, and function of all tagged service and control valves. One copy of each drawing and schedule should be mounted under glass where directed. A copy of each drawing and schedule should also be included as a part of the operations and maintenance manuals. Valve tags shall be at least 40 mm round tags with white characters describing the system and valve designation. Fire protection and fire alarm systems shall be identified as required by NFPA standards and NIH Standard Specifications. Medical/lab gas piping systems should be readily identifiable by appropriate labeling with metal tags, stenciling, stamping, or adhesive markers. Color coding should be used in accordance with NIH Standard Specifications.
top
F.6 Piping Systems
This section is intended to define the general installation requirements for the numerous piping systems installed at the NIH. Many codes govern the actual sizing and installation of piping and should be used during the design process. Welding shall conform to current standards and recommendations of the National Certified Pipe Welding Bureau and all Occupational Safety and Health Administration, State fire protection, and NFPA Standard 241 requirements. Pipe and fittings shall be specified to meet one of the numerous industry standards such as ANSI, ASTM, AWWA, and so on and should be suitable for the operating temperatures and pressures to be encountered on the project. The project engineer, when deemed necessary, shall provide pipe stress analysis to the NIH.
Piping and conduits, except electrical conduits run in floor construction, shall be designed to run parallel with the lines of the building. Electrical conduits shall not be hung on hangers with any other service pipes. The different service pipes, valves, and fittings should be installed so that, after the covering is applied, there will not be less than 13 mm clear space between the finished covering and other work and between the finished covering and parallel adjacent pipes. Hangers on different service lines, running parallel with each other and nearly together, should be in line with each other and parallel to the lines of the building. The minimum pipe size shall be 19 mm for plumbing systems and HVAC systems, and 32 mm for fire protection systems. Size reductions may occur only immediately adjacent to equipment connections. Valves and specialties serving equipment should be full pipe size, not the reduced equipment connection size except where engineering calculations necessitate a different size. Hangers should be spaced to prevent sag and permit proper drainage of piping. Hangers should be spaced not more than 2.4 m apart, unless a greater spacing is specifically designed. A hanger should be placed within 300 mm of each horizontal elbow.
Materials and application of pipe hangers and supports shall conform to the latest requirements of ANSI/ASME B31.1 or ANSI/ASME B31.9 and MSS Standard Practice SP- 58, SP-69, and SP-89, and appropriate Federal specifications where applicable. All materials and anchorage methods for installations in Seismic Zones 3 and 4 shall comply with local building code requirements and shall utilize materials and methods as approved by the local body governing the jurisdiction.
Vertical runs of pipe and conduit less than 4.6 m long should be supported by hangers placed 300 mm or less from the elbows on the connecting horizontal runs. Vertical runs of pipe and conduit over 4.6 m long, but not over 18.3 m long, and not over 150 mm in size, shall be supported by heavy steel clamps. Clamps should be bolted tightly around the pipes and conduits and should rest securely on the building structure without blocking. Clamps may be welded to the pipes and placed below coupling. In lieu of individual hangers, multiple (trapeze) hangers for accessible piping should be considered for water pipes having the same elevation and slope and for electrical conduits. Each multiple hanger should be designed to support a load equal to the sum of the weights of the pipes, conduits, wire, and water and the weight of the hanger itself, plus 90 kg. The structural engineer must approve the structural loads caused by installation of large-diameter piping (200 mm and larger). Safety factors shall be in accordance with ANSI/ASME B31.1. Loading on anchors shall not exceed 25 percent of the proof load test. The size of the hanger rods should be such that the stress at the root of the thread will not be over 68 950 kPa at the design load. No rod should be smaller than 9 mm. The size of the horizontal members should be such that the maximum stress will not be over 103 425 kPa design load. Where vertical piping is specified to extend through sleeves, the riser clamp or pipe support shall transverse the sleeve directly to structure. Allow for differing rates of expansion and contraction of piping systems. Do not anchor piping of substantial operating temperature differences to the same hanger. Trapeze hangers supporting large-diameter piping (200 mm and larger) shall be placed to load joists at top panel points only.
Plastic piping shall be installed to permit proper movement and prevent stresses from expansion and contraction, as well as to protect from damage to piping from abrasion.
Fireproofing shall not be damaged by installation of any hanger or attachment. Where existing fireproofing is disturbed, it shall be restored as approved by the NIH Fire and Safety Officer.
Steam, condensate, and other hot service piping should be designed with loops, bends, and offsets to allow for thermal expansion and keep stresses within the allowable limits of the piping material. Expansion joints or ball joints should be avoided if possible. Rollertype pipe supports should be specified where significant horizontal pipe movement will occur as a result of thermal expansion, and spring-type supports shall be specified where significant vertical movement will occur and where vibration isolation is critical.
Piping should be designed and installed without due stress or strain and run parallel to the lines of the building, except to grade them as specified in a neat and workmanlike manner using a minimum of fittings. Specify provision of thrust restraints to prevent pipe blowout or joint separation due to test procedures or system thrust loads. Such fittings, valves, and accessories should be designed as may be required to meet the conditions of installation and accommodate service. All piping systems, materials, valves, joining methods, and components shall be suitable for the application, location, size, and working pressure of the system at the design operating pressure. Piping should be designed to suit the necessities of clearance with ducts, conduits, and other work and so as not to interfere with any passages or doorways and allow sufficient headroom at all places. No piping should penetrate ductwork.
Gas-piping systems and other hazardous services should be designed in strict compliance with applicable codes and standards. Gas vents, relief valves, rupture disc, and so on shall be piped safely outdoors. Overflow pipes, system drains, and relief devices should be piped to suitable drainage facilities and indirectly connected. Certain pieces of equipment may have high discharge rates that can quickly result in flooding. Drains, sumps, or other receiving devices must have the storage volume required.
Unions or flanges on each side of all pieces of equipment and other similar items should be designed in such a manner that they can be readily disconnected. Union flanges shall be placed in a location that will be accessible after completion of the project.
The project engineer should specify testing procedures in the commissioning guide/plan developed for all components installed on the project. Test procedures should include all items required by code and be sufficient to prove all systems tight at conditions that exceed the maximum design conditions. Water sampling to establish a treatment plan, pipeline sterilization, positive pressure, and vacuum testing may be included as part of the procedures.
Pipe and fittings for NIH buildings shall be as defined in Table F.6.
Table F.6 Pipe Assembly
Service |
Abbreviation |
Color Code |
Pipe |
Fitting |
Joints |
1. |
Sanitary Drainage |
SAN |
Green |
|
|
|
a. |
Underground and aboveground 1. Pipe 375 mm and smaller (except kitchen or grease waste) 2. Pipe 450 mm and larger |
|
|
A A/B1 C |
I I, II III |
a a b |
b. |
Aboveground within building (optional) 1. Pipe 375 mm and larger (except kitchen and grease waste) |
|
|
D |
IV |
c |
c. |
Aboveground within building (optional) (except kitchen, urinal, and grease waste) |
|
|
Q |
VIII |
e |
d. |
Vent piping |
SANv |
|
B |
II |
b |
e. |
Vent pipe (optional) 1. Sanitary vent piping 2. Sanitary vent piping |
SANv |
|
D Q |
IV VIII |
d e |
f. |
Aboveground trap arms and indirect waste |
SANv |
|
D |
VIII |
c |
d. |
Aboveground trap arms and indirect waste (except urinals, water closets, blood analyzers, and corrosive waste) |
SANv |
|
Q |
VIII |
e |
h. |
Sanitary waste for large-animal areas (optional) |
SAN |
|
K |
XIV |
f |
i. |
Underground sanitary trap primer lines |
SAN |
|
R |
XVIII |
e/l |
j. |
Aboveground sanitary trap primer lines |
SAN |
|
R |
XVIII |
e |
k. |
Pumped sanitary waste 100 mm and smaller |
SAN |
|
Q |
XVIII |
e |
l. |
Pumped sanitary waste aboveground and underground 150 mm and larger |
SAN |
|
C |
III |
e |
m. |
Pumped sanitary waste aboveground all sizes |
SAN |
|
AA |
IX |
k |
n. |
Vapor vents from oil interceptors |
SANv |
|
D |
IV |
c |
2. |
Kitchen Waste and Grease Waste |
SAN |
Green |
|
|
|
|
1. Underground 2. Underground (option) 3. Aboveground waste and vent 4. Aboveground waste and vent (option 1) 5. Aboveground waste and vent (option 2) 6. Aboveground vent (option 1) 7. Aboveground vent (option 2) 8. Aboveground exposed waste, traps, and trap arm |
SAN
SAN/SANv |
|
E F B3 E F/G D Q Q4 |
|
g/h I a g/h I d e e |
3. |
Laboratory |
SAN, LW, LV |
Green |
|
|
|
|
1. Underground waste (option 1) 2. Underground waste (option 2) 3. Aboveground waste and vent 4. Aboveground waste and vent (option 1) 5. Aboveground food service area |
|
|
E H J5 E E, I, J6 |
V X XI V V/IX/XII |
g/h m/p n g/h g, h, m, o, p |
a. |
Underground acid waste trap primer lines |
|
|
I, K, L, FF |
XIII |
q |
b. |
Aboveground acid waste trap primer lines |
|
|
I and FF |
XIII |
q |
c. |
Pumped acid waste |
|
|
H |
X |
m |
d. |
Corrosive indirect waste |
|
|
I, K, L, FF |
XIII |
q |
4. |
Photo-Processing Drainage and Vent |
PPD, PPL |
Green |
|
|
|
|
1. Underground waste and vent 2. Underground waste and vent (option 1) 3. Aboveground waste and vent |
|
|
E H7,8 E |
V X V |
g/h m/p g/h |
5. |
Biohazardous Waste and Vent |
BW, BV |
Green |
|
|
|
a. |
1. Underground waste6 2. Underground waste (option 1) 3. Underground waste (option 2) 4. Underground and aboveground waste (option 1) |
|
|
H H J9 M10 |
X X XI XV |
m m n r |
b. |
Aboveground waste (option 1) Aboveground waste (option 2)1 Aboveground waste (option 3)11 |
|
|
N7 N11,7 J12 |
X X XI |
m m/p n |
6. |
Stormwater and Miscellaneous Clearwater Waste |
SW, D |
Green |
|
|
|
a. |
Underground 375 mm and smaller 1. Option 1 2. Option 2 Underground 450 mm and larger |
|
|
A B O1 |
I II XVI |
a a b |
b. |
Aboveground 375 mm and smaller 1. Option 1 2. Option 2 3. Option 3 |
|
|
D A Q |
IV I VIII |
c a e |
c. |
Pumped storm and clearwater drain 100 mm and smaller Pumped storm drain above and below ground 150 mm and larger All sizes |
|
|
Q O AA |
VIII XVI IX |
e j k |
d. |
Stormwater and clearwater drain lines above food service area |
|
|
Q6 |
VIII |
e |
7. |
Foundation Drain/Subsoil Drainage |
FD |
N/A |
P |
XVII |
s |
8. |
Condensate Drain |
CD |
Yellow |
Q |
VIII |
e |
9. |
All Lab Drainage and Vent Piping Above Food Service Areas |
N/A |
Green |
E,I,J |
V,XI, XII |
g/h/o/m/ p |
10. |
Incoming Water Service |
CW |
Green |
|
|
|
a. |
Aboveground incoming service - 65 mm through 100 mm |
|
|
T |
XVIII |
l |
b. |
Aboveground incoming service - 100 mm and larger |
|
|
V |
XIX |
u/v |
c. |
Underground incoming service - 75 mm and larger |
|
|
X |
XXI |
t |
d. |
Underground incoming service - 75 mm and smaller |
|
|
U13 |
XVIII |
l |
11. |
Domestic Cold Water |
CW |
Green |
|
|
|
a. |
Aboveground 65 mm and smaller 75 mm through 200 mm 200 mm and larger 1. Option 1 2. Option 2 |
|
|
T T
W Y |
XVIII XVIII
XX XXII |
w l 2 V r/x |
b. |
Underground 65mm and smaller13 |
|
|
U |
XVIII |
l |
12. |
Domestic Hot Water/Tempered Water |
HW |
Yellow |
|
|
|
a. |
Aboveground 65 mm and smaller 75mm through 150 mm |
|
|
T T |
XVIII XVIII |
W l |
13. |
Drinking Water |
DW |
Green |
T |
XVIII |
w |
14. |
Laboratory Cold Water |
LCW |
Green |
|
|
|
a. |
Aboveground 65 mm and smaller 75 mm through 200 mm 200 mm and larger 1. Option 1 2. Option 2 |
|
|
T T
V Y |
XVIII XVIII
XIX XXII |
w l
u/v r/x |
b. |
Brass nipples and fittings |
|
|
Z14,15 |
XXII |
k |
15. |
Laboratory Hot Water |
LHW |
Yellow |
|
|
|
a. |
Aboveground 65 mm and smaller 75 mm through 200 mm 200 mm and larger 1. Option 1 2. Option 2 |
|
|
T T
Y W |
XVIII XVIII
XXII XX |
w l
r/x v |
b. |
Brass nipples and fittings |
|
|
Z14,15 |
XXIII |
k |
16. |
Laboratory Hot Water Circulating |
LHRW |
Yellow |
|
|
|
a. |
Aboveground 65 mm and smaller 75 mm through 150 mm |
|
|
T T |
XVIII XVIII |
w l |
17. |
Purified Animal Drinking Water |
ADW |
Green |
|
|
|
|
1. Option 1 2. Option 2 |
|
|
BB CC |
XXIV XXV |
y z |
18. |
Softened Water |
SW |
Green |
|
|
|
a. |
Aboveground 65 mm and smaller 75 mm through 150 mm |
|
|
T T |
XVIII XVIII |
z l |
19. |
Deionized Water |
DI |
Green |
DD |
XXVI |
aa |
20. |
Distilled Water |
DIS |
Green |
EE |
XXXII |
bb |
21. |
Reverse Osmosis Water |
ROS/ROR |
Green |
EE |
XXXII |
bb |
22. |
Chilled Water Supply and Return |
CHWS/ CHWR |
Green |
|
|
|
a. |
700 mm and larger |
|
|
MM |
XXIX |
m |
b. |
300 mm through 600 mm |
|
|
KK |
XXIX |
m |
c. |
65 mm through 250 mm |
|
|
ii |
XXIX |
m |
d. |
65 mm to 150 mm (optional) |
|
|
ii Q |
XXXIII |
h d |
e. |
50 mm and smaller |
|
|
ii |
XXVIII |
jk |
f. |
50 mm and smaller (optional) |
|
|
T |
XVIII |
e |
23. |
Secondary Chilled Water Supply and Return |
SCHWS/ SCHWR |
Green |
|
|
|
a. |
300 mm and larger |
|
|
KK |
XXIX |
r |
24. |
Chilled Water Supply and Return |
CHWS/ CHWR |
Green |
|
|
|
a. |
700 mm and larger |
|
|
MM |
XXIX |
r |
b. |
300 mm through 600 mm |
|
|
KK |
XIII |
r |
c. |
65 mm through 250 mm |
|
|
ii |
XXIX |
r |
d. |
65 mm to 150 mm (optional) |
|
|
T |
XVIII |
E/l d |
e. |
50 mm and smaller |
|
|
ii |
XXVIII |
k |
f. |
50 mm and smaller (optional) |
|
|
T |
XVIII |
e |
25. |
Secondary Chilled Water Supply and Return |
SCHWS/ SCHWR |
Green |
|
|
|
a. |
300 mm and larger |
|
|
KK |
XXIX |
r |
b. |
125 mm through 250 mm |
|
|
ii |
XXIX |
r |
c. |
100 mm and smaller |
|
|
T |
XVIII |
e |
26. |
Glycol Water Supply and Return |
GWS/GWR |
Green |
|
|
|
a. |
65 mm and larger |
|
|
ii |
XXIX |
r |
b. |
65 mm to 125 mm (optional) |
|
|
ii |
XXXIII |
ff |
c. |
50 mm and smaller |
|
|
ii |
XXVIII |
k |
d. |
50 mm and smaller (optional) |
|
|
KK |
XVIII |
e |
27. |
Condenser Water Supply and Return |
CWS/CWR |
Green |
|
|
|
a. |
300 mm and larger |
|
|
KK |
XXIX |
r |
b. |
65 mm through 250 mm |
|
|
ii |
XXIX |
r |
c. |
65 mm and larger (optional) |
|
|
ii |
XXXIII |
ff |
28. |
Cooling Water Supply and Return (Process Cooling) |
CS/CR |
Green |
|
|
|
a. |
125 mm and larger |
|
|
ii |
XXIX |
r |
b. |
100 mm and smaller |
|
|
T |
XVIII |
e |
29. |
Heating Water Supply and Return |
HWS/ HWR |
Yellow |
|
|
|
.a. |
300 mm and larger |
|
|
KK |
XXIX |
r |
b. |
65 mm through 250 mm |
|
|
ii |
XXIX |
r |
c. |
50 mm and smaller |
|
|
ii |
XXVIII |
ff |
d. |
50 mm and smaller (optional) |
|
|
T |
XVIII |
e |
e. |
50 mm and smaller (optional) |
|
|
T |
XVIII |
e |
30. |
Heat Recovery Supply and Return |
HRS/HRR |
Yellow |
|
|
|
a. |
300 mm and larger |
|
|
KK |
XXIX |
r |
b. |
65 mm through 250 mm |
|
|
ii |
XXIX |
r |
c. |
50 mm and smaller |
|
|
ii |
XXVIII |
k |
d. |
50 mm and smaller (optional) |
|
|
T |
XVIII |
e |
31. |
Secondary Heating Water Supply and Return |
SHS/SHR |
Yellow |
|
|
|
a. |
300 mm and larger |
|
|
KK |
XXIX |
r |
b. |
125 mm through 250 mm |
|
|
ii |
XXIX |
r |
c. |
100 mm and smaller |
|
|
T |
XVIII |
e |
32. |
Steam Supply (175 psi Maximum) |
HPS/ MPS/LPS |
Yellow |
|
|
|
a. |
300 mm and larger |
|
|
KK |
XXIX |
r |
b. |
65 mm through 250 mm |
|
|
ll |
XXIX |
r |
c. |
50 mm and smaller |
|
|
ll |
XXXIV |
k |
33. |
Steam Vents |
SV |
Yellow |
|
|
|
a. |
65 mm and larger |
|
|
ii |
XXIX |
r |
b. |
50 mm and smaller |
|
|
ii |
XXXIV |
k |
c. |
50 mm and smaller (optional) |
|
|
ii |
XXXV |
r |
34. |
Steam Condensate |
HPR/ MPR/LPR |
Yellow |
|
|
|
a. |
65 mm and larger |
|
|
JJ |
XXIX |
r |
b. |
50 mm and smaller |
|
|
JJ |
XXXIV |
k |
c. |
50 mm and smaller (optional) |
|
|
JJ |
XXXV |
r |
35. |
Pump Condensate |
PC |
Yellow |
|
|
|
a. |
65 mm and larger |
|
|
JJ |
XXXVI |
r |
b. |
50 mm and smaller |
|
|
JJ |
XXXIV |
k |
c. |
50 mm and smaller (optional) |
|
|
JJ |
XXXV |
r |
36. |
Steam Instrumentation |
SI |
Yellow |
|
|
|
a. |
50 mm and smaller |
|
|
ii |
XXXIV |
k |
b. |
50 mm and smaller (optional) |
|
|
ii |
XXXV |
r |
37. |
Blow-Down |
BD |
Yellow |
|
|
|
a. |
50 mm and smaller |
|
|
ii |
XXXIV |
k |
b. |
50 mm and smaller (optional) |
|
|
ii |
XXXV |
r |
38. |
Feedwater |
FW |
Yellow |
|
|
|
a. |
65 mm and larger |
|
|
ii |
XXIX |
r |
b. |
50 mm and smaller |
|
|
ii |
XXXIV |
k |
c. |
50 mm and smaller (optional) |
|
|
ii |
XXXV |
r |
39. |
Makeup Water |
MW |
Green |
T |
XVIII |
e |
40. |
Oxygen |
O2 |
Yellow /Blue |
|
|
|
a. |
Aboveground |
|
|
GG |
XXVII |
cc |
b. |
Underground |
|
|
HH16 |
XXVII |
cc |
41. |
Nitrogen and Vent |
N2 |
Green/ Black |
|
|
|
a. |
Standard pressure |
|
|
GG |
XXVII |
cc |
b. |
High pressure |
|
|
HH |
XXVII |
cc |
42. |
Nitrous Oxide and Vent |
NO2 |
Black/ Blue |
GG |
XXVII |
cc |
43. |
Carbon Dioxide and Vent |
CO2 |
Yellow |
|
|
|
a. |
Aboveground |
|
|
GG |
XXVII |
cc |
b. |
Underground |
|
|
HH16 |
XXVII |
cc |
44. |
Helium |
HE |
Brown |
GG |
XXVII |
cc |
45. |
Medical Air |
MA |
Yellow |
GG |
XXVII |
cc |
46. |
Medical Vacuum |
MV |
White |
GG17 |
XXVII |
l |
47. |
Laboratory Air |
LA |
Yellow |
GG |
XXVII |
cc |
48. |
Laboratory Vacuum and Vent |
LV |
Yellow |
Q |
XVIII |
l |
49. |
Gas Evacuation |
GE |
Yellow |
GG |
XXVII |
l |
50. |
Animal Air |
AA |
Yellow |
GG |
XXVII |
cc |
51. |
Animal Vacuum |
AV |
Yellow |
GG17 |
XXVII |
i |
52. |
Animal Oxygen |
O2A |
Yellow/ Blue |
GG |
XXVII |
cc |
53. |
Acetylene/Acetylene Mixtures |
C2H2 |
Yellow |
ii |
XXVII |
l |
54. |
Fuel Supply |
FOS/FOR |
Yellow |
|
|
|
a. |
65 mm and larger |
|
|
ii |
XIII |
r |
b. |
50 mm and smaller |
|
|
ii |
XV |
k |
55. |
Fuel Oil Vent |
FOV |
Yellow |
ii |
XIII |
r |
56. |
Natural Gas |
NG |
Yellow |
|
|
|
a. |
Aboveground 14 kPa (2 psi) and less, size 65 mm and smaller 14 kPa (2 psi) and less, size 75 mm and larger 35 kPa (5 psi) and greater Underground – outside building Exposed fume hood and laboratory Equipment connections |
|
|
ii18
ii18 ii18 N
ii/oo |
XXVIII
XXIX XXIX XXX XXVIII XXXI |
k
r r dd
k/ee |
57. |
Compressed Air19 |
CA |
Blue |
Q,T,G G HH |
XVIII XXVII |
l |
58. |
Dental Vacuum |
DV |
Yellow |
|
|
|
a. |
Aboveground and underground 1. Option 1 2. Option 2 |
|
|
Q K |
VIII XIV |
e f |
59. |
Refrigerant Piping |
RS/RL |
Yellow |
|
|
|
a. |
65 mm and larger |
|
|
ii |
XXIX |
m |
b. |
50 mm and smaller |
|
|
T |
XVIII |
gg |
60. |
Refrigerant Relief |
RR |
Yellow |
ii |
XXIX |
r |
61. |
Generator Exhaust |
N/A |
N/A |
ii |
XXIX |
r |
Notes:
- Hub may be cut off below ground and extra heavy pipe extended above grade for appropriate transition adapter to aboveground material. (This allows pipe to fit in standard walls and chases.)
- Insulate lines from condensation. Provide with double containment, PVC, or flame-retardant polypropylene, minimum thickness of SDR-33, watertight joints to 10 psi.
- Do not use this material for waste lines from soda fountains.
- Do not use for floor sink and floor drain traps or trap arms.
- Glass shall not be used for vents through roof.
- Provide PVC or flame-retardant polypropylene double containment, minimum thickness of SDR-33, and capable of 10 psi.
- Not recommended for BSL-4.
- Provide double containment, minimum thickness SDR 33 polypropylene or PVC. Not recommended for BSL-4.
- Provide with double containment, minimum SDR 33 polypropylene or PVC. Provide with leak monitoring cable for BSL-4.
- Provide double containment, minimum Schedule 5, ASTM A312 Type 316 Schedule 5 Stainless Steel. Provide with leak monitoring cable for BSL-4.
- Provide with double containment, minimum SDR 33 polypropylene or PVC. Provide with leak monitoring cable.
- Provide with double containment and leak monitoring cable for BSL-4. Glass shall not be used for vents through roof.
- No joints permitted below building slab.
- NIPPLES: Red Brass Schedule 40, ANSI B687, regular or extra strong.
- Fittings: CHROME PLATING for finished locations: ASTM B456.
- Provide continuous schedule 40 PVC sleeve, with minimum 100 mm concrete encasement and metallic warning tape at sleeve and again halfway between initial backfill and finished grade.
- The use of uncleaned pipe and fittings shall be permitted only where special variance is granted in response to an acceptable plan presented by the contractor to prevent possible misuse of the vacuum system materials on a medical gas system.
- Pipe and fittings shall be provided with protective coating for installation in corrosive environments and exterior installations, Skotchkote, Greenkote epoxy coating or minimum two coats rust-inhibiting paint.
- Select as required based on system application, pressure, and cleanliness requirements.
F.6.1 Piping Material:
Pipe material designation indicated in Table F.6, Pipe Assembly, shall conform to the specifications in Table F.6.1.
Table F.6.1 Piping Material Specifications
Designation |
Pipe Specifications |
A |
Cast iron hub and spigot pipe, service weight, ASTM A74. |
B |
Cast iron hub and spigot, extra heavy weight, ASTM A74. |
C |
Ductile iron Class 53, ASTM A746 with fusion-bonded epoxy or ceramic epoxy interior lining. |
D |
Cast iron hubless pipe, ASTM A74, ASTM A888, CISPI 301. |
E |
High silicon iron pipe, ASTM A518, ASTM A861. |
F |
Stainless steel waste pipe, Type 316L, ANSI A112.3.1. |
G |
Stainless steel waste pipe, Type 304L, ANSI A112.3.1 (added product). |
H |
Polypropylene pipe, ASTM F1412, ASTM D4101, ASTM D618, ASTM D2447 Schedule 80. |
I |
Flame retardant polypropylene pipe, ASTM F1412, ASTM D4101, ASTM D618, ASTM D635, ASTM D2843, Schedule 40 (added proper standards for this material). |
J |
Borosilicate glass pipe, ASTM C1053. |
K |
Polyvinyl chloride pipe, ASTM D1785 or ASTM D2665 dual stamped Schedule 40. Cellular core or foam core not accepted. |
L |
Polyvinyl chloride pipe, ASTM D1785 Schedule 80. |
M |
Stainless steel pipe, ASTM A312, Type 316 Schedule 40, seamless. |
N |
Flame retardant polypropylene pipe, ASTM F1412, ASTM D4101, ASTM D618, ASTM D2447, ASTM D635, ASTM D2843. |
O |
Ductile iron pipe, Class 53, ASTM A746. |
P |
Porous concrete pipe, AASHTO M-176. |
Q |
Seamless copper tube, ASTM B88, Type L Hard. |
R |
Seamless copper tube, ASTM B88, Type L Soft. |
S |
Not used (reserved) |
T |
Seamless copper tube, ASTM B88, Type K Hard. |
U |
Seamless copper tube, ASTM B88, Type K Soft. |
V |
Ductile iron pipe, cement lined, ANSI/AWWA C150, C151, AWWA C104, ANSI A21.4, Class 53, Universal Phenolic exterior with TNEMEC 37H-77 primer, all materials NSF- 61 compliant. |
W |
Ductile iron flanged pipe, cement lined, ANSI/AWWA C150, C151, AWWA C104, ANSI A21.4, AWWA C115, Class 53, suitable for operation to 212 °F, Universal Phenolic exterior with TNEMEC 37H-77 primer, all materials NSF-61 compliant. Piping for hot water shall not have sealcoat. |
X |
Ductile iron pipe, cement lined, ANSI/AWWA C150, C151, AWWA C104, ANSI A21.4, Class 53, AWWA C105 8-mil polyethylene encasement. |
Y |
Stainless steel pipe, Type 316L, Schedule 10 or Schedule 40, ASTM A312, ASTM A778. Schedule 40 should be utilized for systems with low water velocities. Minimum 0.61 m/s velocity for any stainless steel piping system. |
Z |
Brass pipe and nipples ASTM B43, ANSI B687 seamless regular or extra-strong, ASTM B456 chrome plating for finished locations. |
AA |
Galvanized steel pipe, ASTM A53 or ASTM A106, Grade B, Type S, ASTM A123/A153 Schedule 40. |
BB |
CPVC pipe, ASTM F441, Class 23447B, Type IV, Grade 1, Schedule 40 or Schedule 80. |
CC |
Stainless steel sanitary tube, ASTM A270, ASTM A450, ANSI B36.19M, Schedule 10 electropolished 130 to 150 grit sanitary interior. |
DD |
Unpigmented polypropylene pipe, ASTM D4101, ASTM D2837, SDR 11, individual cap, sealed bag. |
EE |
As required by program requirements and required water quality. |
FF |
PVC, polypropylene, or stainless steel tubing as required by application (added product). |
GG |
Copper tube, cleaned and degreased for oxygen service, Type L hard, nitrogenized and ends capped. Any piping contaminated or not under nitrogen charge at time of installation not accepted. Type ACR not accepted. |
HH |
Copper tube, cleaned and degreased for oxygen service, Type K hard, nitrogenized and ends capped. Any piping contaminated or not under nitrogen charge at time of installation not accepted. Type ACR not accepted. Provide continuous sleeve and encasement for underground medical gas piping as directed under Piping Section. |
ii |
Black steel pipe, ASTM A53 or A106, (for generator exhaust ASTM A53 is not applicable) Grade B, Type S seamless Schedule 40. |
JJ |
Black steel pipe, ASTM A53 or A106 Grade B, Type S seamless Schedule 80. |
KK |
Carbon steel pipe, ASTM A53 or A106 (for high pressure steam ASTM A53 is not applicable) Grade B, Type XS, extra heavy wall seamless. |
LL |
Carbon steel pipe, ASTM A106, Grade B, Type XS extra heavy wall, seamless. |
MM |
Carbon steel pipe, API-5L Type DSAW, Grade B, 0.5 inch wall thickness double submerged arc-welded longitudinal seam. |
NN |
Polyethylene fuel gas tube, ASTM D2513, ASTM 1784, Type II, Class B, Category 3, Grade P24. Minimum thickness of SDR 11. |
OO |
Steel fuel gas tubing, ASTM A539 electric resistance welded. |
F.6.2 Fitting Materials:
Fitting material designations indicated in Table F.6, Pipe Assembly, shall conform to the specifications in Table F.6.2.
Table F.6.2 Fitting Materials Specifications
Designation |
Specifications |
I |
Service cast iron hub and spigot, ASTM A74. |
II |
Extra heavy cast iron hub and spigot, ASTM A74. |
III |
Ductile iron, Class 53 ASTM A746 fusion-bonded epoxy or ceramic epoxy interior lining, drainage pattern. |
IV |
Cast iron hubless fittings, CISPI 301. |
V |
High silicon iron drainage pattern fittings. |
VI |
Type 316 stainless steel, drainage pattern, ANSI A112.3.1. |
VII |
Type 304 stainless steel, drainage pattern, ANSI A112.3.1. |
VIII |
Wrought copper and bronze drainage pattern fittings, ANSI B16.23, B16.29, or ANSI/ASME B16.32. |
IX |
Galvanized cast iron, Durham drainage pattern, ASTM A126, ANSI B16.12, ASTM A153. |
X |
Polypropylene drainage pattern, ASTM F1412, ASTM D4101, ASTM D618, ASTM D2447, ASTM D3311. |
XI |
Borosilicate glass drainage pattern, ASTM C1053. |
XII |
Flame-retardant polypropylene drainage pattern, ASTM F1412, ASTM D4101, ASTM D618, ASTM D2843, ASTM D635, ASTM D2447, and ASTM D3311. |
XIII |
Chemically resistant compatible with system pipe material and application. |
XIV |
Polyvinyl chloride, drainage pattern, ASTM D2665. |
XV |
Stainless steel ASTM A312 Type 316 Schedule 40 constructed to equivalent ASTM D3311 drainage fitting patterns. |
XVI |
Ductile iron, Class 53 drainage pattern fittings, ASTM A746. |
XVII |
Porous concrete drainage fittings, ASTM C654, AASHTO M176. |
XVIII |
Wrought copper solder cup type fittings, ANSI/ASME B16.22 or B16.18. |
XIX |
Ductile iron cement lined, ANSI/AWWA C110, or C153, AWWA C104/ANSI A21.4, special thickness Class 53 or 1 724 kPa, Universal Phenolic exterior with TNEMEC 37H-77 primer, all materials NSF-61 compliant. |
XX |
Flanged ductile iron cement lined, ANSI/AWWA C110, or C153, AWWA C115, AWWA C104/ANSI A21.4, special thickness Class 53 or 1 724 kPa, Universal Phenolic exterior with TNEMEC 37H-77 primer, all materials NSF-61 compliant. Fittings for hot water shall not have seal coat. |
XXI |
Ductile iron cement lined, ANSI/AWWA C110, or C153, AWWA C104/ANSI A21.4 special thickness Class 53 or 1 724 kPa, all materials NSF-61 compliant, AWWA C105 8-mil polyethylene encasement. |
XXII |
Stainless steel mechanical groove joint, Type 316L, Schedule 10 or Schedule 40, ASTM A312, ASTM A778. |
XXIII |
Cast bronze pressure threaded pressure fittings, ANSI B16.5. Provide with ASTM B456 chrome plating for finished locations. |
XXIV |
CPVC Schedule 40 or Schedule 80, ASTM F439 Type IV, Grade I socket type, Schedule 80 threaded type. |
XXV |
Stainless steel sanitary fitting, Type 316, Schedule 10, electropolished sanitary interior 130 to 150 grit, ASTM A270, ASTM A450, ANSI B36.19M. |
XXVI |
Natural polypropylene, unpigmented ASTM D4101, ASTM D2839, furnished in sealed nitrogen-charged bag. |
XVII |
Wrought copper solder cup type fittings, ANSI/ASME B16.22, factory cleaned and degreased for oxygen service. Factory nitrogenized and bagged, maximum 20 fittings per bag. |
XXVIII |
Black malleable iron threaded fittings, ANSI B16.3 for less than 517 kPa and 136 kg for 517 kPa or more. |
XXIX |
Steel butt weld fittings, ANSI B16.9, ASTM A234, long turn ells, ANSI B16.5 weld-neck or slip-on flanges and Bonney Forge Weldlets and threadlets, wall thickness to match pipe. |
XXX |
Polyethylene fuel gas fittings, ASTM D2683, ASTM D2513, Type II, Class B, Category 3, Grade P24, ASTM D1248 butt fusion or socket fusion. |
XXXI |
Swaglock fuel gas fitting to match piping application. Use only for final equipment connection at fume hoods and similar equipment. |
XXXII |
As required by program requirements and required water quality. |
XXXIII |
Black malleable iron grooved fittings and couplings, ASTM A47. |
XXXIV |
Black cast iron threaded fittings, ANSI B16.4, 57 kg less than 517 kPa and 113 kg for 517 kPa and more. Steam condensate shall be 113 kg for all pressures. |
XXXV |
Black steel socket weld fittings, ANSI B16.11, wall thickness to match pipe. |
XXXVI |
Galvanized steel grooved fittings and couplings, ASTM A47M or ASTM A536. Note: Cut-groove type only. Roll-grooving not permitted for galvanized piping. |
F.6.3 Joint Materials:
Joint material designations indicated in Table F.6, Pipe Assembly, shall conform to the specifications in Table F.6.3.
Table F.6.3 Joint Material
Designation |
Joint Material |
a |
Premolded neoprene compression gasket to ASTM C564. |
b |
Compression gasketed (Tyton) joints or mechanical joints. |
c |
Heavy-duty shielded couplings meeting CISPI 310, with stainless steel shield and neoprene gasket. Couplings shall be minimum of 4-band type, unless FM1680 approved otherwise. |
d |
CISPI 310 stainless steel shielded neoprene coupling. |
e |
ASTM B32 lead-free soldered joints, noncorrosive flux. |
f |
Solvent cemented ASTM 2564 NSF listed cement and ASTM F656 NSF listed primer to IAPMO installation standard 9-95. “Hot” cements or wet-type fast dry cements shall not be utilized. Solvent cement joints only in dry ambient conditions, and cement type appropriate for ambient temperature and pipe size. (These full criteria important due to common poor installation and potential joint failures.) |
g |
Hub and spigot caulked type with acid resistant packing and molten lead in accordance with manufacturer requirements. |
h |
Mechanical joint type with Teflon seal, neoprene outer gasket, and stainless steel shield as provided by pipe and fitting manufacturer. |
i |
Elastomeric sealed socket type joint by pipe and fitting manufacturer. |
j |
Neoprene gasketed lock-ring type restrained joints by pipe and fitting manufacturer. |
k |
Threaded using American Standard for Pipe Threads, ANSI B2.1 with thread sealant or Teflon tape material especially listed compatible with system contents, pipe materials, and operating conditions. |
l |
BCUP 2, 3, 4, or 5 brazed joints. |
m |
ASTM D2657 socket fusion to practice method 1. |
n |
Mechanical joint type with Teflon seal, neoprene gasket, and stainless steel shield over bead to bead or bead to plain end where required. |
o |
SASTM F1290 electrofusion with stainless steel coil. |
p |
ASTM D2657 butt fusion method. |
q |
To match pipe material. |
r |
Butt weld to ANSI B31.1 and MCAA Part VII, Standard Procedure Specification Parts 1 and 2. |
s |
Tongue and groove, mortar sealed. |
t |
AWWA C111 mechanical joint with restraint fitting/retainer gland or approved neoprene gasketed restraint push on joint. Full restraint and thrust blocks. All bolts, nuts, and accessories AWWA compliant grade and type, Cor-Blue bolts and nuts. |
u |
AWWA cut groove method with NSF-61 listed gasket. |
v |
AWWA C115 flanged with AWWA C111 special gasket type, EPDM. |
w |
ASTM B32 lead-free solder with ASTM B813 water soluble flux. |
x |
Mechanical groove joint with galvanized steel or stainless steel coupling, NSF-61 listed EPDM gasket. |
y |
ASTM D2846, F-493 NSF listed solvent cemented joints with ASTM F656 NSF listed primer. |
z |
Sanitary butt weld, interior bead removed, or sanitary mechanical joint (clean joint). Infrared weld (IR butt fusion) or crevice-free butt weld. |
aa |
As required by program requirements and required water quality. |
bb |
BCUP 2, 3, 4, or 5 brazed joints without flux to NFPA 99 Level 1 system standards and ASSE series 6000 installation procedure, including clean, dry nitrogen purge. |
cc |
ASTM D2657 socket fusion or butt fusion only. Mechanical joints shall not be used. |
dd |
Swaglock fitting only at final connection to equipment. |
ee |
ASTM A183 coupling, nuts, and bolts, ASTM D2000 rubber gaskets for water service. |
ff |
AWS A5.8 Bag-5 with AWS Type 3 flux, except Type BcuP 5 or BcuP 6 may be used for copper to copper joints. |
gg |
Methods and materials for wet taps, where permitted by the NIH, should be submitted for approval by the A/E. Submittals should include documentation on the products to be used with complete instructions and procedures to ensure successful wet taps. |
top
F.7 Insulation Systems
Insulation should be applied to mechanical systems to limit heat loss, prevent condensation, protect people from hot or extremely cold surfaces, and improve the operating efficiency of all systems. The value of proposed insulation systems should be justified by comprehensive life-cycle costing and present-worth analysis. Insulation materials approved for use in NIH buildings should have a fire hazard rating not to exceed 25 for flame spread and 50 for fuel contributed and smoke developed. All materials should be factory tested as an assembly. Fire ratings should be determined by the standard method of testing for surface-burning characteristics of building materials, ASTM E84 or NFPA Standard 255. Insulation approved for use shall have a UL label or a certified test report from an approved testing laboratory. Insulation materials should not be installed on systems until all necessary tests have been conducted for each component and insulated surfaces have been thoroughly cleaned and are in a dry state.
All adhesives, sealers, vapor barrier coatings, and so on used in conjunction with insulation should be compatible with the material to which they are applied. Any cement, sealer or coating used should be resistant to vermin and mold. All insulation surfaces should be durable and, where exposed, protected from damage due to maintenance operations, vandalism, weather, and normal wear and tear. Preformed insulation systems are preferred at pumps, valves, strainers, and access doors for ease of maintenance and to lower cost. Protective jackets consisting of 0.23 kg canvas or 0.41 mm aluminum shall be used for exposed insulation systems.
Pipe fittings and valves, where possible, should be protected using factory- premolded fittings, covers, and factory-protect insulation. Large valves and specialties should be protected using custom-made canvas jackets with straps and buckles to allow frequent removal and reinstallation without damaging the jacket. Metallic components used for the installation of insulation systems should be suitable for the intended environment and should not corrode. Exposed external corners on duct and equipment insulation in occupied areas shall be protected by corner beads consisting of 50 x 50 x 0.41 mm-thick aluminum.
Insulation systems should be specified to meet industry standards, and installation requirements shall, as a minimum, include the following:
- Insulation should be continuous at all hangers, hanger rods, supports, sleeves, and openings. Vapor seals must be provided for all cold surfaces and should be continuous. Where supports must occur below the insulation surface, the thickness shall be maintained over the support and extend sufficiently beyond the support to prevent condensation. Insulation should be sealed where it terminates because of a valve, union, flange, and so on.
- All insulation should be arranged to permit expansion and contraction of systems without causing damage to the insulation or surface.
- The actual insulation thickness must be at least equal to the minimum specified at all locations, including supports in contact with cold surfaces.
- It is critical that insulation materials be installed in a first-class manner with smooth and even surfaces. Scrap pieces of insulation should not be permitted where a full-length section will fit.
- High-density pipe saddles or welded pipe standoffs should be provided at all points of pipe support.
- All valves and strainers shall be insulated, and premolded covers and factory-precut insulation or custom-fabricated jackets should be used where applicable. Unions and flanges shall not be insulated except on cold services.
- Valves should be insulated up to and including bonnets, except for cold water valves, which should be insulated over packing nuts in a manner to permit removal for adjustment and repacking.
- Strainers should be insulated to permit removal of the basket without disturbing the insulation of the strainer.
- On ductwork or equipment, accessories should be provided as required to prevent distortion and sagging of insulation. Welded pins, adhesive clips, and wire ties should be provided as recommended by the manufacturers and SMACNA.
- Duct and equipment insulation should cover all standing seams and metal surfaces with full-thickness insulation.
- Cold-water pumps should be insulated with removable and replaceable square or rectangular covers consisting of full 1.3 mm gauge aluminum metal jackets reinforced at corners and edges and lined with insulation. Pumps with split casings should be constructed with insulated housing in two or more sections with the upper section removable for access to the casing. Cover sections should be flanged, gasketed, and joined with stainless steel sheet metal screws. Lube fittings and drain valves should extend outside insulated covers.
- Where deemed necessary by the A/E, specific insulation details should be added to the contract documents to improve the insulation performance on the project.
Insulation may be omitted on the following items at the discretion of the A/E:
- Brass or copper pipe specified to be chrome plated (typically applies to toilet rooms)
- Traps and pressure-reducing valves, concealed relief piping from safety valves, and unions, flanges, and expansion joints on hot piping systems
- All fire protection and fuel piping
- Exposed ducts in air-conditioned spaces
- Existing adjacent insulation on alteration projects
- ASME stamps
- Access plates of fan housings
- Cleanouts or handholes
- Components within factory-preinsulated HVAC equipment
- Factory-preinsulated flexible ductwork
- Factory-preinsulated HVAC equipment
- Manufacturer’s nameplates
- Vibration-isolating connections
F.7.1 Pipe Insulation:
Table F.7.1 defines the minimum insulation standards for NIH projects and is intended as a guide for the services listed and other similar services not indicated. The A/E should select the most suitable product for each individual service.
Table F.7.1 Insulation Material for Piping
Service |
Material |
Specifications |
Type |
Class |
Vapor Barrier |
Chilled water (supply and return, dual temperature piping, 4 °C nominal) |
Cellular Glass Urethane |
ASTM C552 ASTM C591 |
II |
2 |
Yes Yes |
Hot domestic water supply and recirculating piping (maximum 93 °C) |
Cellular Glass Urethane Mineral Fiberglass |
ASTM C552 ASTM C591 ASTM C547 |
II I |
2 |
No Yes Yes |
Cold domestic water piping above and below ceilings |
Cellular Glass Urethane Mineral Fiberglass |
ASTM C552 ASTM C591 ASTM C547 |
II I |
2 |
Yes Yes Yes |
Heating hot water (supply and return, maximum 121 °C) |
Calcium Silicate Cellular Glass |
ASTM C533 ASTM C552 |
I II |
2 |
No No |
Refrigerant suction piping (177 °C nominal) |
Flexible Cellular Mineral Fiber |
ASTM C534 ASTM C547 |
I |
1 |
Yes Yes |
Compressed air discharge, steam and condensate return (94 to 121 °C) |
Cellular Glass Mineral Fiber Calcium Silicate |
ASTM C552 ASTM C547 ASTM C533 |
II
I |
1 |
No No No |
Drinking fountain, drain piping (to sewer tie-in) |
Mineral Fiber Cellular Glass Flexible Cellular |
ASTM C547 ASTM C552 ASTM C534 |
II I |
1 2 |
Yes Yes Yes |
Exposed lavatory drains, exposed domestic water piping and drains to areas for persons with disabilities |
Preformed Fire Retardant, Antifungal, Closed Cell Foam Insulation Kit |
ASTM C534 |
I |
|
No |
Horizontal interior storm drain and overflow drain piping, including transition fitting from vertical to horizontal, and underside of roof and overflow drain bodies |
Mineral Fiber |
ASTM C553 |
I |
B-3 |
Yes |
A/C condensate drain located inside building |
Mineral Fiber Cellular Glass Flexible Cellular |
ASTM C547 ASTM C533 ASTM C534 |
II I |
1 2 |
Yes Yes Yes |
Medium-temperature hot water, steam and condensate (122 to 177 °C) |
Calcium Silicate Cellular Glass |
ASTM C533 ASTM C534 |
I I or II |
|
No No |
Brine systems cryogenics (- 30 to 0 °C) |
Cellular Glass Polyisocyanurate |
ASTM C552 ASTM C591 |
II |
2 |
Yes Yes |
Brine systems cryogenics (0 to 34 °C) |
Cellular Glass Polyisocyanurate |
ASTM C552 ASTM C552 |
II |
2 |
Yes Yes |
F.7.2 Piping Insulation Thickness:
Table F.7.2 Piping Insulation Thickness
Service |
Material |
Tube and Pipe Size (mm) |
6-32 |
40-80 |
90-125 |
150-250 |
280-400 |
Chilled water (supply and return) and dual temperature piping) (4 °C nominal) |
Cellular Glass Cellular Glass Urethane Polyisocyanurate |
40 40 20 25 |
50 40 20 25 |
50 40 20 25 |
65 40 25 25 |
80 40 25 25 |
Hot domestic water supply and recirculating piping (maximum 93 °C) |
Cellular Glass Urethane Polyisocyanurate |
40 20 25 |
40 20 25 |
40 25 25 |
40 25 25 |
40 25 25 |
Cold domestic water piping above and below ceiling |
Cellular Glass Flexible Cellular Urethane Polyisocyanurate |
40 15 20 25 |
40 15 20 25 |
40 15 20 25 |
40 N/A 25 25 |
40 N/A 25 25 |
Heating hot water (supply and return, maximum 121 °C) |
Calcium Silicate Cellular Glass |
50 40 |
50 40 |
65 40 |
65 40 |
65 40 |
Refrigerant suction piping (177 °C) |
Flexible Cellular Cellular Glass |
15 40 |
N/A 40 |
N/A 40 |
N/A 40 |
N/A 40 |
Compressed air discharge, steam and condensate return (94 to 121 °C) |
Mineral Fiber Calcium Silicate Cellular Glass |
40 50 40 |
50 80 40 |
65 100 40 |
80 100 40 |
90 115 40 |
Exposed lavatory drains, exposed domestic water piping and drains to areas for handicapped personnel |
Flexible Cellular |
15 |
15 |
15 |
16 |
16 |
Horizontal roof drain leaders (including underside of roof drain fitting) |
Mineral Fiber |
25 |
25 |
40 |
40 |
50 |
A/C condensate drain located inside building |
Mineral Fiber Cellular Glass Flexible Cellular |
20 40 15 |
25 40 15 |
25 40 15 |
25 40 N/A |
25 40 N/A |
Medium-temperature hot water and steam (122 to 177 °C) |
Calcium Silicate Cellular Glass |
65 40 |
90 40 |
115 40 |
115 50 |
125 65 |
High-temperature (177 to 204 °C) and steam (177 to 260 °C) |
Calcium Silicate Cellular Glass Composite |
65 25 50 |
80 25 50 |
100 25 50 |
100 25 50 |
100 25 50 |
Brine systems cryogenics (34 to 0 °C) |
Cellular Glass Polyisocyanurate |
65 35 |
65 35 |
80 45 |
80 45 |
80 45 |
Brine systems cryogenics (0 to 171 °C) |
Cellular Glass Polyisocyanurate |
50 30 |
50 30 |
50 30 |
65 35 |
80 45 |
F.7.3 Duct Insulation:
Table F.7.3.a Minimum Duct Insulation Thermal Resistance Factors
DELTA to °C |
27 |
54 |
81 |
108 |
136 |
162 |
R-Value |
1.8 |
3.6 |
5.4 |
7.2 |
9.1 |
11.1 |
Note: Duct systems, or portions thereof, shall be insulated to provide a thermal resistance, excluding film resistances, of
R = DELTA t (m2·h·°C/J) = 15
Where DELTA t = design temperature differential between air in the duct and the duct surface in degrees centigrade. Noninsulated ducts in noninsulated sections of exterior walls and in attics above the insulation might not meet requirements of ASHRAE Standard 90A. Required thermal resistances do not consider condensation. Additional insulation with vapor barriers may be required to prevent condensation under some conditions. For residential buildings with uninsulated roofs over attics containing ducts, air temperatures shown in Table F.7.3.b should be used. Excerpted by permission from ASHRAE Standard 90A, published by American Society of Heating, Refrigerating and Air-Conditioning Engineers, Atlanta, Georgia.
Table F.7.3.b Residential Attic Temperature
Roof Pitch: Summer Conditions |
°C |
5 in 12 and up |
54 |
3 in 12 to 5 in 12 |
60 |
Less than 3 in 12 |
66 |
Roof pitch: winter conditions |
|
All roof pitches |
12° greater than outdoor design temperature |
Reference: Excerpted by permission from ASHRAE Standard 90A, published by American Society of Heating, Refrigerating and Air-Conditioning Engineers, Atlanta, Georgia.
F.7.4 Insulation Materials for Equipment:
Table F.7.4.a Insulation Materials for Equipment
Equipment |
Spec. |
Type |
Class |
Flexible mineral fiber Surface temperatures up to 204 °C |
ASTM C553 |
1 |
B-3 |
Rigid mineral fiber Surface temperatures up to 204 °C Surface temperatures up to 454 °C |
ASTM C612 |
|
1 or 2 3 |
Cellular glass surface temperatures between -240 and +427 °C |
ASTM C552 |
1, 2, or 3 |
1 or 2 |
Table F.7.4.b Insulation Thickness for Equipment
Equipment |
Recommended Thickness (mm) |
Expansion tanks or pneumatic water tanks |
25 |
Air separators |
50 |
Pumps |
50 |
Hot water storage tanks |
50 |
Heat exchangers, such as steam-to-hot-water convertors Up to 121 °C 121 to 204 °C 205 to 316 °C |
50 90 150 |
Hot water duct-mounted coils |
50 |
Chilled water tanks * 177 to 13 °C |
25 |
Cryogenic Equipment * -34 to -17 °C |
100 |
* Vapor barrier is required.
F.7.5 Insulation for Boiler Stack and Diesel Engine Exhaust Pipe:
Table F.7.5 Insulation and Thickness for Boiler Stack and Diesel Engine Exhaust Pipe
Service and Surface Temperature Range (°C) |
Material |
Outside Diameter (mm)) |
6-32 |
40-80 |
90-125 |
150-250 |
280-900 |
Boiler stack (up to 204) |
Mineral Fiber ASTM C553, Class B-3, ASTM C547, Class 1, or ASTM C612, Class 1 |
N/A |
N/A |
80 |
90 |
100 |
|
Calcium Silicate ASTM C533, Type 1 |
N/A
|
N/A |
80 |
90 |
100 |
|
Cellular Glass ASTM C552, Type II |
40
|
40
|
40
|
50 |
65 |
Boiler stack (205 to 316) |
Mineral Fiber ASTM C547, Class 2, ASTM C592, Class 1 or ASTM C612, Class 3 |
N/A |
N/A |
100 |
100 |
125 |
|
Calcium Silicate ASTM C533, Type 1 |
N/A |
N/A 40 |
100 |
100 |
100 |
|
Mineral Fiber/Cellular Glass Composite:
Mineral Fiber ASTM C547, Class 2 ASTM C592, Class 1 or ASTM C612, Class 3
Cellular Glass ASTM C552, Type II |
25
50 |
25
50 |
25
50 |
25
50 |
50
50 |
Boiler stack (316 to 427) |
Mineral Fiber ASTM C547, Class 3 ASTM C592, Class 1 or ASTM C612 Class 3 |
N/A |
N/A |
100 |
100 |
150 |
|
Calcium Silicate ASTM C533, Type 1 |
N/A |
N/A |
100 |
100 |
150 |
|
Mineral Fiber/ Cellular Glass Composite:
Mineral Fiber ASTM C547, Class 2 ASTM C592, Class 1 or ASTM C612, Class 3
Cellular Glass ASTM C552, Type II |
50
50 |
50
50 |
50
50 |
80
80 |
100
50 |
Diesel engine exhaust (up to 371) |
Calcium Silicate ASTM C533, Type I |
80 |
90 |
100 |
100 |
100 |
|
Cellular Glass ASTM C592, Type II |
65 |
90 |
100
|
115 |
150 |
top
F.8 Testing and Balancing
The A/E for NIH buildings should specify a complete and comprehensive system operation that includes testing, adjusting, and balancing of environmental and other systems to produce the design objectives. The testing and balanced shall be done in coordination with commissioning as required by the NIH Model Commissioning Guide. Air conditioning as defined by ASHRAE is the process of treating air to control simultaneously its temperature, humidity, cleanliness, and distribution to meet the comfort requirements of the conditioned space. The balance process serves as the quality control function to ensure that the air-conditioning systems are performing to the specified design intent.
Each air treatment process in the conditioning system contributes a specific function to produce proper environmental conditions. However, it is the coordinated action of all these processes in a system that produces the desired effect. If any one of these coordinated functions does not perform to expectations, the final results will affect the overall system performance. The balancing process must confirm that the entire system produces the results for which it was designed.
The testing and balancing (TAB) process shall be specified to meet one or both of the following standards (latest edition):
- National Standards for Total System Balance as defined by the Associated Air Balance Council (AABC) and/or
- Procedural Standards for Testing, Adjusting and Balancing of Environmental Systems as published by the National Environmental Balancing Bureau (NEBB)
All work must be performed by an independent TAB contractor who is an approved member of either the AABC or NEBB.
The TAB process should be specified to meet the National Standards for Total System Balance as defined by the AABC’s latest edition or NEBB. All work provided must be performed by an independent TAB contractor who is an approved member of the AABC or NEBB. The TAB contractor should provide all required pre-construction plan checks and reviews; should test, adjust, and balance the air and water system; and should submit completed reports, floor plans indicating TAB points, analysis, and verification data showing proper system performance that meets the intent of design.
TAB is a science that requires understanding of design intent, proper use of instruments, evaluation of readings, and adjustment of the system to design conditions. The mere ability to use an instrument does not qualify a person as a TAB engineer or technician. Qualification requires training and years of field experience in applying proven techniques and analyzing gathered data. Project specifications should require that only trained engineers and technicians be allowed to test and balance systems in NIH buildings.
NIH building projects often are constructed and occupied in multiple phases. The TAB process specified should address the requirements of interim balancing to support partial occupancy of buildings. The health and safety of occupants and the environmental conditions must be suitable for continuous operation at all phases during construction.
Mechanical systems may require complete or zone balancing at the end of each phase. Once fully occupied, entire systems may require reverification to ensure that later phases have not created deficiencies in early ones. Multiple drive assemblies may be required for fans. Followup TAB work may also be required during premium time to avoid disruption in research functions.
The completion of TAB services seldom occurs smoothly because of construction problems and occupancy functions, equipment malfunction, or institute-supplied equipment not in the scope of work. The A/E, Project Officer, Division of Safety, commissioning agent, and research staff should develop an occupancy/move plan and operation strategy that can be specified in the contract documents and force the TAB process to accommodate its intended requirements. The project engineer’s task will be to define a generalized TAB procedure that fully supports the phasing and clearly ties down the full scope of work at bid time. All costs associated with TAB services through the completion of building occupancy should be included at bid time.
top
F.9 Program Equipment
The selection and use of program equipment such as refrigerators, freezers, centrifuges, autoclaves, glassware washers, biological safety cabinets, fume hoods, and so on should be established early in the design phase so that mechanical and electrical systems can be designed to support specific equipment requirements. All equipment selected for use in NIH facilities should meet NFPA, OSHA, NSF, and NIH Fume Hoods Specifications requirements. Equipment selected should not contain asbestos, lead, and mercury. The A/E shall carefully ascertain equipment requirements so that heat rejection, electrical usage, and other utility consumption data are defined for system design. Spatial requirements for equipment must be closely reviewed, and layouts should allow for access to all piping, wiring, and ductwork connections and easy cleaning and replacement of parts. Mechanical systems should be designed and detailed so that they do not induce harm to or impede the operating efficiency of program equipment. Pressure regulators, safety relief valves, gravity drainage facilities, temperature controls, and backflow protection devices should be provided as required to protect equipment.
Program equipment as defined by the program of requirements should be connected to an independent energy management and control system to monitor integral equipment alarms. The complete control and operation/maintenance strategy for program equipment should be closely reviewed against program requirements and with building occupants. The maintenance of such equipment often dictates the magnitude of control points and monitoring facilities.
top
F.10 Motors and Drives
Motors and drive assemblies should be selected to optimize the efficiency of mechanical and building systems. Motors must always be of adequate size to drive the equipment without exceeding the nameplate rating at the speed specified or at the load that may be obtained by the drive.
Motors shall be rated for continuous duty at 115 percent of rated capacity and base temperature rise on an ambient temperature of 40 °C. Motors 560 W and larger shall be three-phase, Class B, general-purpose, squirrel cage, open-type, high-efficiency induction motors in accordance with National Electrical Manufacturers Association (NEMA) Design B standards, wound for voltage specific to the project, 60 Hz AC, unless otherwise required by the design. Motors smaller than 560 W shall be single- phase, open-capacitor type in accordance with NEMA standards for 115 V, 60 Hz, AC. Motors 124 W and smaller may be the split-phase type.
All motors utilized on NIH projects shall have the minimum efficiency as scheduled below. Nameplate rating and efficiency shall be per Institute of Electrical and Electronics Engineers (IEEE), Test Procedure 112, Method B:
Table F.10 Minimum Motor Efficiency
Motor Size |
0.75 |
1.1 1.5 |
2.2 3.7 |
5.6 7.5 |
11.2 14.9 |
18.7 22.4 |
29.8 37.3 |
44.8 |
56.0 |
74.6 93.3 |
112.0 - 149.2 |
Min. kW |
82.5 |
84.0 |
87.5 |
89.5 |
91.0 |
92.4 |
93.0 |
93.6 |
94.1 |
94.5 |
95.0 |
All motors 0.75 kW and larger should have a composite power factor rating of 90 to 100 percent when the driven equipment is operating at the design duty. Devices such as capacitors, or equipment such as solid-state power factor controllers, should be provided as part of the motor or motor-driven equipment when required for power factor correction.
Variable speed drives (VSD) of various types should be employed on NIH projects to vary the flow of water and air. The A/E should evaluate the specific application of each speed drive and provide life-cycle costing to prove its economic viability. Other variable-flow devices such as inlet vanes may be considered for smaller systems.
The A/E should consider the following issues when employing VSDs for NIH buildings:
- When main and standby equipment is to be controlled by speed drives, only one drive shall be provided to serve both pieces of equipment. Consider providing VSD as part of motor control center (MCC).
- Equipment motors should be matched to the drive so that low speeds can be realized.
- Speed drives shall have a manual bypass completely independent of the drive cabinet. Motors shall operate at full speed in the bypass position when the speed drive is deenergized and open for service.
- When deemed necessary because of the critical nature of equipment served, multiple drives may be provided for redundant equipment.
- The level of reliability required of the VSD system should be identified.
- The operational overloads and starting conditions required by the application shall be defined. Typical requirements may be: variable torque = 115 percent for 1 minute; constant torque = 150 percent for 1 minute.
- The way control commands for the VSDs will be generated by the process should be determined, i.e., manual/potentiometer analog current loop, 4-20 mA serial communication (RS232, RS485, etc.) Isolated or nonisolated process feedback (pressure, temperature, flow, etc.)
- The characteristic surges, sags, or momentary discontinuities present in the supply and any other nonlinear loads on the feeder should be defined.
- The levels of voltage distortion on the power system should be determined before the VSD is applied. Before-and-after distortion effects on the supply system should be evaluated and defined about the harmonic current spectrum injected into the system and its effect on the other loads.
- The levels of voltage on the power system before the VSD is applied; the harmonic current spectrum to be injected into the supply system by the VSD, the magnitude of distortion on the supply voltage before and after, and whether this harmonic current injection will affect other loads shall be defined.
- What speed range is required and whether the load will be operated beyond base speed range should be defined.
- It shall be determined whether all parts of the rotating load are suitable for the range of vibration excitation frequencies.
- The waveform the VSD produces and whether there are any constraints on the motor connections’ length should be determined.
- It shall be verified that the motor is sized to provide the necessary load torque while operating at reduced speed. The power capability of the motor may be restricted at low speeds. The motor output capability should be compared with the load requirement. An additional cooling fan may be required for constant torque loads. (This pertains to constant torque systems, such as compressors, etc.)
- The heat rejection from the VSD controller and how the losses are removed from the equipment should be defined. The heat generated with the VSD is normally removed by air or water cooling.
- The range of voltage and frequency of the electric supply that will permit full rated output of the VSD should be defined. What happens outside the range, what line transients can be tolerated, and what the VSD input power factor is should be considered.
- How the VSD operates under fault conditions should be defined, for example, a mechanical overload, an electrical short circuit in the motor circuit, or a ground fault in the load system.
- The motor protection provided by the VSD equipment and any additional protection required for comprehensive system protection, e.g., overload, overspend, reverse rotation, should be defined.
- The manufacturer should be required to submit information for system operations and maintenance and to sell diagnostic, warranty, training, and operation and maintenance manuals.
- The total power factor (PF) (i.e., real PF and apparent PF) should be defined. The difference between the two is caused by inductance (reactive element) in transformers, motors, etc.
F.10.1 Harmonic Voltage and Currents: Variable frequency drives (VFDs) typically inject harmonic currents into the power system as a result of the nonlinear nature of switching in electronic power devices. The harmonic currents combine with the system impedance frequency response characteristic and create harmonic voltage distortion.
Several concerns associated with harmonic distortion levels need to be addressed in the project specification. This will avoid significant harmonic-related problems with both the VFD equipment and the NIH operations controlled. These concerns include the following:
- Harmonic distortion on both the supply and motor side of the drive
- Equipment de-rating due to harmonic distortion produced by VFDs
- Audible noise caused by high-frequency (several kHz) components in the current and voltage
- Harmonic filter design and specifications
Where VFDs are appropriate for use, 18 pulse VFDs shall be provided for all motors 75 horsepower and above. For motors less than 75 horsepower, 6 or 12 pulse VFDs with harmonic filters (passive or active), phase multiplication devices, or any other components required to mitigate harmonic voltage total harmonic distortion (THD) to 5% and current THD to 3% at full load / 5% at any load maximum levels shall be an integral part of the variable frequency drive system. Compliance measurement shall be based on actual THD measurement at the VFD circuit breaker terminals during full load VFD operation. Designs which employ shunt tuned filters shall be designed to prevent the importation of outside harmonics which could cause system resonance or filter failure. Calculations supporting the design, including a system harmonic flow analysis, shall be provided as part of the submittal process for shunt tuned filters. Any filter designs which cause voltage rise at the VFD terminals shall include documentation in compliance with the total system voltage variation of plus or minus 10%. Documentation of Power Quality compliance shall be part of the commissioning required by the VFD supplier. Actual job site measurement testing shall be conducted at full load and documented in the operation and maintenance manuals. Harmonic measuring equipment utilized for certification shall carry a current calibration certificate. The final test report shall be reviewed and compliance certification stamped by a licensed professional engineer. Text and graphical data shall be supplied showing voltage and current waveforms, THD and individual harmonic spectrum analysis in compliance with the above standards.
Control wiring for VFDs shall NOT be run in same conduit as power conductors.
F.10.2 Voltage Sag Concerns: Despite the main advantages provided by VSDs, the concern for nuisance tripping during voltage sag conditions remains. This power quality concern involves the control sensitivity to short-duration voltage sags and momentary interruptions. Actually, many different kinds of controls, and even motor contractors, are sensitive to these voltage sags. Voltage sags caused by faults on the power system represent one of the most important problems that can be experienced by the NIH with sensitive loads. Whenever there is a fault on the transmission or distribution system serving the NIH facility (faults cannot be completely avoided regardless of the system design), there will be either a voltage sag or an interruption. If the fault occurs on a parallel-distribution feeder circuit or on the transmission system, there will be a voltage sag that lasts until the fault is cleared by some protective device (typically 3-30 cycles depending on the fault location). A method of predicting the likelihood of faults in a certain region along with knowledge of equipment sensitivity can be used to determine an “area of vulnerability.” A combination of computer short-circuit simulations and lightning performance analysis should be used to determine the affected area. The VSD controls should be designed to handle these voltage sag conditions without tripping. The specifications contain no-ride-through capability. This is an important consideration when VSDs are applied in critical processes such as that of the NIH, where nuisance tripping can cause significant problems. The designer should evaluate the level of sensitivity of the controls to voltage sags. If such concern exists, applying power conditioning to the controls themselves will be considered. Feroresonant transformers can handle voltage sags down to approximately 60 percent of the nominal voltage. This is sufficient to handle virtually all voltage sags caused by single line-to-ground faults on the power system. If additional protection is needed, the controls can be protected with an uninterruptible power supply (UPS) system, which can handle complete interruptions in the input signal.
F.10.3 Transient Overvoltage Concerns: Transient overvoltage occurs in con-nection with capacitor switching. Each time a capacitor is energized, a transient voltage oscillation occurs between the capacitor and power system inductance. The result is a transient overvoltage that can be as high as 2.0 V per unit (of the normal voltage) at the capacitor location. The magnitude is usually less than 2.0 V per unit as a result of dampening provided by system loads and losses. The transient overvoltage caused by capacitor energizing is generally not a concern to PEPCO because its magnitude is usually below the level at which surge-protective devices operate (1.5 to 2.0 V per unit). However, these transients can be magnified at the NIH facility if the NIH has low-voltage capacitor banks for (displacement) power factor correction. The designer should check for this matter. When the frequency of a transient overvoltage matches the series-resonant frequency of the NIH’s transformer coupled with PEPCO capacitor(s) at the East Substation, a low-impedance, high-current (at the resonant frequency) condition results. As this large current passes through the NIH’s transformer, it induces a large voltage “drop” that passes through zero voltage to create a large voltage of opposite sign (because of a phase-angle change) at the resonant frequency.
The VSD and the NIH’s paralleled capacitor (and their surge protection devices) then see this magnified voltage (compared to distribution feeder voltage). When the resonant-frequency current completes its path to ground through the capacitor, the voltage experiences a “boost” to the ground-reference voltage. The magnification of capacitor-switching transients is most severe when the following condition exists: The capacitor switch on the higher voltage system is much larger (kVAR) than the capacitor at the low-voltage bus. Generally, this situation occurs most frequently for substation switching. The frequency of oscillation that occurs when the high-voltage capacitor is energized is close to the resonant frequency formed by the stepdown transformer in series with the low-voltage capacitor. There is little resistive load on the low-voltage system to provide dampening of the transient, as is usually the case for industrial plants (motors do not provide significant damping of these transients). It is not uncommon for magnified transients at low-voltage capacitors to range from 3.0 to 4.0 V per unit. These transients have significant energy associated with them and are likely to cause failure of protective devices, metal oxide varistors (MOVs), electronic components (silicon-controlled rectifiers, etc.), and capacitors. VSDs are particularly susceptible to these transients because of the relatively low peak-inverse voltage ratings of the semiconductor switches and the low energy ratings of the MOVs used to protect the VSD power electronics. The following should be evaluated and identified in the specifications to control these magnified transient overvoltages: using vacuum switches with synchronous closing controls to energize the capacitor bank and control the capacitor-switching transient; providing high-energy MOV protection on the 480 V buses (the energy capability of these arresters should be at least 1 kJ); or using tuned filters for power factor correction instead of just shunt capacitor banks (the tuned filters change the frequency response of the circuit and usually prevent magnification problems; this solution combines power factor correction, harmonic control, and transient control).
F.10.4 Electromagnetic Interference and Radio Frequency Interference Concerns: IEEE Standard 519, Recommended Practices and Requirements for Harmonic Control in Electric Power Systems, recommends limits for voltage distortion and harmonic current resulting from nonlinear loads. However, the IEEE standard is not intended to cover the effects of radio frequency interference (RFI). As a result, specifications will occasionally refer to Federal Communications Commission (FCC) Rules and Regulations, Volume 2, Part 15, Subpart J, Class A (referred to as “FCC rule”) to establish limits on electromagnetic emission for VSDs. The FCC rule was printed in October 1982 primarily for computing devices. Computers generate RF energy and possibly cause interference with nearby equipment if misapplied. Generally, the rule sets conducted and radiation RF limits for electronic devices using timing signals or digital techniques with pulse rates in excess of 10 000 pulses per second. Technically speaking, VSDs with high-frequency timing circuits conform to this description, although they are not intended as a computing device described in the FCC rule. The primary and more significant source of electromagnetic interference (EMI) from a VSD stems from the power circuits, and, in this respect, drives become an incidental radiation device.
The only requirement for incidental radiation devices in the FCC rule is that they shall be operated so that the RF energy emitted does not cause harmful interference. If so, the operator must eliminate the interference. All VSDs, regardless of the manufacturer, will produce electromagnetic emission to some degree. Primarily, these emissions are due to the steep wave fronts and very rapid switching of power semiconductors in the VSD. Typically this occurs when transistors, GTOs, or other “fast devices” are gated on and off in DC chopper circuits and inverter power circuits for PWM, current source, and six-step drives. Typically, conductors to the VSDs and motor act as an antenna and radiate the RF energy into the media. Therefore, it is possible for RF to be induced into nearby antennas and other conductors and be carried to the loads in that circuit. Holding a portable AM radio near a power outlet in close proximity to an EMI source can be evidence of this situation. Distributive digital control (DDC) systems, medical alarms system and equipment, telecommunication services, and other electronic equipment utilizing very high frequencies may experience noisy interference or malfunctions when subject to EM/RF energy.
The specification should clearly outline the corrective measures required. The first and foremost corrective measure to avoid problems associated with EMI is proper routing of the drive conductors in separate metallic conduits (even separate raceways if practical) as remote as possible from any other conductors or suspect equipment. Usually, this will be sufficient to avoid EMI problems. EM/RF filters can be engineered for a system to trap or inhibit high-frequency emissions into power system conductors. However, because of the nature of EMI, the effectiveness of any filter is highly sensitive to where it is installed. Further, it is not certain that the filter will correct the problem even though it may meet FCC limits. Most manufacturers will include this footnote with their literature: “Filters are expensive and usually require additional space. It is recommended that they be furnished only when they are specifically required to avoid or solve a problem after exhausting all proper installation methods. In addition, filters are an additional component and must be considered in the overall reliability of a power system.” To contain RF radiation through the media from the VSD, complete shielding using a metallic enclosure generally is required. This will usually contain most of the radiated RF to a reasonable distance.
top
F.11 HVAC Systems
The HVAC systems at NIH buildings are highly diverse and must satisfy a large variety of program requirements. The challenge to the HVAC designer is to accurately define systemoperating parameters and to control strategies, heat load data, utility requirements, and program equipment needs. The design engineer and the maintenance engineer must take a proactive role in the early design stages so that operating requirements are defined clearly and concisely. HVAC systems must fully support the program of requirements, utilize stateof- the-art, efficient and proven technology, and promote the health and safety of building occupants. Proposed system alternatives must be evaluated fairly, with consideration given to operating and maintenance cost, reliability, flexibility, redundancy, future expansion capacity, and the value of lost research in the event of system failures. The health and safety of building occupants drive the need for good indoor air quality, and all system alternatives must fully comply with the requirements of these guidelines.
F.11.1 All-Air Systems: In air systems, the air supplied to the spaces provides the cooling and heating capacity necessary to produce the desired temperature, filtration, ventilation, and humidity levels for comfort or process control. The fan energy required for the distribution of the air can be quite significant and is dependent upon the quantity of the air, pressure drops in the conditioning equipment and ductwork, fan and drive efficiencies, and hours of operation. Although ventilation for reduction of contaminants may govern frequently in labs, animal spaces, and special spaces, the quantity of air is usually determined by and is proportional to the space-sensible cooling or heating load and inversely proportional to the difference between room and supply air temperatures. Consequently, reduction in the space-cooling load through prudent design of the building envelope and lighting will produce a reduction in air volume and hence a reduction of the required energy consumption. Fan energy consumption should be optimized through the design of the conditioning equipment, selection of components, and duct design.
Air-handling equipment including intake and exhaust louvers, filters, and heating and cooling coils can be optimized by selection at a conservative face velocity. Lower face velocities can be justified by life-cycle cost analysis. Filter life may be improved by reducing face velocity, permitting an economically justifiable lower final pressure drop (before replacement).
Simpler, shorter duct systems designed with conservatively low duct velocities are consistent with energy efficiency objectives and offer acoustical benefits. High-loss fittings, such as mitered elbows, abrupt transitions, takeoffs, and internal obstructions should be avoided. Long duct runs, if necessary, should be designed with special consideration of pressure loss since the maximum loss for any run should be imposed upon the entire fan system. Duct systems should be designed at the lowest pressure possible given the physical restrictions within buildings.
Air systems should serve spaces having similar operating characteristics. Spaces with different periods of occupancy or substantially different ventilation requirements should not be combined on the same system. Dedicating air systems to specific departments provides proper grouping of spaces with similar occupancy characteristics and environmental performance requirements and simplifies the duct distribution systems.
The use of cold air distribution may be considered for an energy conservation method. However, the A/E must address several factors to ensure that the system successfully distributes the cold air. Cold air distribution systems supply air for space comfort conditioning at nominal temperatures between 3 and 11 °C, as opposed to conventional supply temperatures of 13 and 15 °C. This approach has been applied primarily to take full advantage of the 1 to 3 °C chilled water available with ice storage.
The A/E should assess the full economic impact of the system, such as first-cost reductions that come from the decreased size of air handlers, fans, ducts, chilled water pumps, and piping. In new buildings, there will be structural cost savings due to the decreased floor-tofloor height requirements of smaller ducts. First costs may increase for cooling coils, terminal units, duct insulation, and the cooling storage system, but the net effect may be a reduction in total system costs. Typically, air and water distribution cost reduction of 15 to 20 percent, depending on the size of the system, can be achieved by lowering the supply air temperature from 13 to 7 °C. The net total cost reduction, including refrigeration and storage equipment costs, are typically 6 to 11 percent when comparing a conventional chiller system with 13 °C supply air and an ice storage system with 7 °C supply air.
Systems using nominal 7 °C supply air (range of 6 to 8 °C) should be emphasized because they offer the greater benefits for application with ice storage. The nominal 3 °C supply air (lower than 5 °C) should not be recommended because of the requirement of more specialized equipment and design effort, with little additional savings.
Very often overlooked parameters in the economic impact are:
- The cooling energy, which increases with cold air distribution because more dehumidification is performed than is required for room comfort.
- The penalty for the reduced availability of economizer cooling.
The A/E should consider that at the typical relative humidity (RH) levels of 35 to 45 percent, dry-bulb temperature can be increased -17 to -16 °C above conventional room comfort set points, and occupants will perceive the same comfort conditions. This effect can result in a 5 to 10 percent reduction in cooling energy and in most cases is critical to achieving a net energy reduction with cold air distribution. The optimum supply air temperature should be determined by an economic analysis that considers changes in first costs and operating costs for various design options.
A blow-through configuration, with the cooling coil downstream of the supply fan, should be utilized for the cold air distribution systems. This allows the fan heat to be absorbed directly in the coil, resulting in a supply air temperature -17 to -16 °C lower than for a draw-through configuration. Coils with 8 to 12 rows should be used to provide supply air at 6 to 8 °C. Fin spacing should not exceed 12 fins per square meter, and the fin configuration should allow easy cleaning.
A high chilled-water temperature rise in the coil is recommended to reduce pumping horsepower and to increase the efficiency of refrigeration equipment operating in chiller mode. In general, a -7 °C rise can easily be achieved with storage systems. A 4 °C rise is recommended for cold air distribution systems, and in some cases a rise as high as -1 °C.
The coil face velocity should not be more than 1.778 m/s-2.286 m/s (350-450 fpm), with a maximum limit of 2.286m/s (450 fpm). A low face velocity requires a large coil and airhandling unit but achieves better coil heat transfer and a lower supply air temperature. A higher face velocity results in smaller equipment but is limited by carryover of moisture from the coil into downstream ductwork. The coil face velocity for heating only units may approach 2.54 m/s (500 fpm).
The A/E should be aware of oversizing of coils with ice storage systems that can lead to problems and should approach with care. Excessive dehumidification and poor control of chilled water flow can result, along with overcooling of the space, if the air side is also oversized.
Round ducts should be used for the cold air distribution. If round ducts cannot be used, the aspect ratio (ratio of width to height) of rectangular ducts should be minimized to reduce pressure losses and initial costs.
The minimum insulation thickness for a cold air distribution system will be determined by requirements for preventing condensation. The optimum thickness, which will likely be greater than this minimum, is determined by an economic analysis of the cost of additional insulation versus the penalties from duct heat gained and increased supply-air temperature. These penalties include the increased size of equipment and additional energy consumption.
A direct cold air supply requires the use of diffusers that will perform satisfactorily with the expected supply air temperatures, especially at low loads. The design must be such that colder, low-velocity air will not dump directly into the space. Before designing such a system, the diffuser manufacturer must be consulted and tests run to ensure that the diffuser will perform adequately with the expected supply air temperature. Long supply-air duct runs can have a temperature rise of -15 to -13 °C at low airflow. In such cases, the duct temperature rise at low loads may prevent dumping for systems with supply air as low as 6 °C at the cooling coil.
F.11.2 Air and Water Systems: Air and water systems should be composed of a central ventilation system and four pipe-fan coil units. The system should utilize both chilled water and hot water piping to each terminal fan coil unit.
Controls for room fan coil units should be sequenced to avoid simultaneous heating and cooling with provisions for an adjustable dead band between cooling and heating modes, unless relative humidity control is essential, in which case simultaneous cooling and heating may be considered.
Fan coil units may be utilized to provide supplemental cooling for equipment areas or other spaces with large internal heat gains and limited ventilation requirements. The central air systems should be utilized in conjunction with the fan coil units to maintain minimum ventilation rates. Induction-type terminal units should not be utilized. Secondary pumps designed for the heating or cooling piping loops should be automatically controlled to shut off when their function is unnecessary.
Shutoff gate valves should be used in all heating, glycol, water, industrial hydronic systems, steam, and condensate lines (recommended use of OS&Y 300# ANSI for high pressure and 150# ANSI for medium and low-pressure system). Bronze stemmed gate valves manufactured by Grinnel, Vale and Jenkins are recommended for use at the NIH campus. All valve stems on systems to be insulated shall be provided as extended type as required to permit sufficient clearance for proper operation without damaging insulation.
High-performance butterfly valves may be used for chilled water, domestic, or industrial cold water services. Gate valves are preferred, and the use of butterfly valves for different applications should be evaluated by the project engineer in consultation with the Resource Management Section of the Public Works Branch. Full ported top entry or three piece bronze, stainless steel ball valves may be used on all water and gas services for sizes 50 mm or less.
F.11.3 Unitary Equipment: The use of unitary equipment should be restricted to serve unique areas, such as computer rooms and support facilities, or as required to maintain specific environmental conditions.
F.11.4 Sound Lining: Duct lining is not permitted for use in duct systems. Omission of duct lining usually requires sound attenuators in order to meet the specified NC criteria. A sound analysis should be performed to ascertain the need of terminal sound attenuators. Sound attenuators should be selected for low velocities and low pressure losses. High-velocity selection should be avoided because of the pressure loss and internally generated noise. Sound attenuators shall have approved lining material to prevent insulation fibers from becoming airborne.
F.11.5 Plenums: The use of plenums or air shafts for air distribution (supply, return, or exhaust) is prohibited in NIH buildings. Common outside ducts may be permitted for multiple air intakes to air-handling units because of the constraints on space and building configuration. Corridors, exit passageways, stairways, and other similar spaces should not be used as plenums or transfer air paths as defined by NFPA and the International Building Code.
F.11.6 Indoor Air Quality: Providing acceptable indoor air quality (IAQ) in NIH buildings is the responsibility of the A/E. These guidelines define acceptable IAQ levels and recommend methodologies to achieve those levels. Typical contaminant control measures include dilution and effective ventilation, local exhaust ventilation, air cleaning, temperature, and humidity control.
The use of mechanical ventilation to maintain relatively comfortable and odor-free indoor spaces is based on the principles of general dilution theory. By doubling the volume of air available for dilution under static or constant conditions of contaminant generation, a 50 percent reduction in contaminant concentration can be expected. If the air volume is doubled again, the concentration will be reduced to 25 percent of its original value, and so on. The converse is also true. By reducing the air volume available for dilution, contaminant concentrations would be expected to correspondingly increase.
However, dilution ventilation is not as effective in many cases. In those instances where contaminants such as formaldehyde and volatile organic compounds (VOCs) are released from sources by diffusion, emission will vary in response to changes in environmental conditions such as temperature, relative humidity, and ventilation rates themselves. Increased ventilation rates, for example, produce vapor pressure exchanges around sources, which cause emission rates to increase. This phenomenon has been reported for formaldehyde and a number of VOCs. With VOCs, a sixfold increase in the ventilation rate will result in a twofold increase in source strength.
The shortcomings of dilution ventilation should be fully addressed by the A/E.
The relationship between CO2 generation rates, occupant density, and outdoor ventilation rates has been well established and serves as the basis for the ASHRAE Standards and WHO guidelines for acceptable IAQ. Because bioeffluent levels determined from CO2 measurements have a relatively constant generation rate for a given occupant density, increased actual outdoor ventilation rates have a predictable effect on bioeffluent levels in buildings. Effects of high and low outdoor ventilation rates as well as occupant density on CO2 should be evaluated.
F.11.7 Air-Handling Systems for Laboratory Buildings: Laboratory buildings should be designed with “once through,” 100 percent outdoor air systems that automatically compensate for filter loading. Laboratory air shall not be recirculated. Systems should have pressure-independent hot water terminal reheat devices and individual laboratory module and/or office area temperature zone control. The HVAC system shall be designed to maintain the proper temperature, humidity, differential pressure, outdoor air exchange rate, and acoustic criteria within the space. Laboratory building air systems should operate continuously year-round. The HVAC system capacity should be based on the larger of the two main parameters specified below:
- The amount of fume hood exhaust required to meet actual design requirements or the minimum exhaust requirement set by chemical fume hood density policy at the NIH, whichever is greater. If the required exhaust to meet actual fume hood demand is less than the air exhaust required to meet minimum hood density requirements of one nominal 1.2 m-wide vertical fume hood (354 L/s) for every other laboratory module, then the fume hood exhaust demand shall be based on NIH Fume Hood Density Policy.
- The required space cooling loads. This is primarily a function of thermal transmission, solar loads, associated laboratory support equipment, and lighting loads. At the NIH, a combined laboratory equipment and lighting load density of 118 W/nm2 shall be used as a minimum in design of laboratory areas (86 W/nm2 for equipment, 32 W/nm2 for lighting).
The ventilation rate should also be sufficient for the removal of contaminate. The following equation provides a single relationship that makes it possible to calculate the steady state, equilibrium concentration that would be produced in a room (or any enclosed space for which the overall volume can be determined) by the complete evaporation of some specific volume of any identifiable volatile solvent.
C = |
|
Vs p T |
|
x (6.24 x 107) |
|
|
[MWs] Patm Vroom |
|
|
Where:
C = equilibrium concentration of volatile solvent that would be produced in the room by evaporation of the known volume of solvent, C measured in ppm.
Vs = volume of solvent that has evaporated, measured in mL.
p = density of solvent, measured in g/cm3; T = temperature in room, measured in °K.
T = temperature in room, measured in °K.
MWs = molecular weight of solvent.
Patm = ambient barometric pressure prevailing in room, measured in mm Hg.
Vroom = volume of room, measured in L.
6.24 x 107 = proportionality constant that makes this equation valid, under NTP conditions.
The following equation is known as the basic room purge equation. It provides the necessary relationship for determining the time required to reduce a known initial high-level concentration of any vapor—existing in a defined closed space or room—to a more acceptable ending lower level concentration.
Dt = |
V |
In |
Cinitial |
Q |
Cending |
Where:
Dt = time required to reduce the vapor concentration in the closed space or room, as required, measured in minutes.
V = volume of closed space or room, measured in m3.
Q = ventilation rate at which the closed space or room will be purged by whatever airhandling system is available for that purpose, measured in m3/s.
Cinitial = initial high-level concentration of vapor in the ambient air of the closed space or room, which concentration is to be reduced—by purging at qm3/m—to a more acceptable ending lower level concentration, measured in ppm.
Cending = desired ending lower level concentration of the vapor that is to result from the purging effort in the closed space or room, also measured in ppm.
The application of the above equations can provide required L/s for dilution and assess fume hood stack concentrations that cannot be used to calculate the reentrainment of contaminated air into outdoor air intake which is referred to in the guidelines. The guidelines identify the use of ASHRAE methodology to locate intakes and stack outlets. However, ASHRAE does not have any guidelines on the required dilution rates. Therefore, the methodology identified by ASHRAE cannot be used without a set dilution requirement.
It is recommended that the reentry be assessed on the basis of the spill of a volatile compound. The spill area will be the fume hood pan (0.813 m2 with Reynolds number of 30 000). From this information, the diffusivity in the air, mass transfer, and stack coefficient can be evaluated and then, by using ASHRAE methodology with a 1 000-rate dilution, the reentry concentrations can be calculated.
The use of the above equations can be used in situations such as the following: a 1.50 L glass bottle full of acetone (MW = 58.08; density = 0.891 g/cm3; and vapor pressure = 226 mm Hg at 25 °C) is spilled from its position on a shelf, breaking when it hits the floor of a lab that has a volume of 47 m3. If all the acetone evaporates, what will the ultimate ambient concentration of acetone be in the room? Is it reasonable to assume that all the acetone will evaporate? To solve the final part, both Dalton’s law of partial pressures and Raoult’s law will have to be applied to the solution obtained in the first part. To solve the first part, the above equations are used:
C = |
Vs p T |
x (6.24 x 107) |
|
[MWs] Patm Vroom |
|
C = |
(1.5) (1 000) (0.79) (273.16 + 25) |
x (6.24 x 107) = 10 702 ppm |
|
(58.08) (760) (1 650) (28.32) |
|
The ultimate ambient concentration level of the acetone vapors in this room will be 10 702 ppm. According to Raoult’s law, the partial vapor pressure of any volatile component will be the product of the vapor pressure of the pure component and the mole fraction of that component in the solution being considered. In this case, dealing with pure acetone, the mole fraction will be 1.00 = 100 percent and Raoult’s law shows that the “potential” for the partial vapor pressure of acetone would simply be its pure state vapor pressure, or 226 mm Hg. Applying Dalton’s law of partial pressures to this fact:
Cmax = |
(1 000 000) (PVPacetone) |
|
Pambient |
Cmax = |
(1 000 000) (226) |
= 297 368 |
|
760 |
|
It is reasonable to assume that all the acetone will evaporate, since the concentration that would be produced by the quantity of acetone in the bottle that was broken during the fall is only a small fraction of the theoretical maximum ace-tone concentration that could exist in the ambient air (10 702 ppm vs. 297 368 ppm, with the former being only 3.6 percent of the latter). In fact, in a room for the size given, 41.7 L of pure acetone could reasonably be expected to evaporate completely. From this, one calculates the fume hood stack concentration and required L/s for purge. For example, if the goal is to ventilate the room until the acetone concentration is at or below the TLV-TWA concentration, which for acetone is 750 ppm, and if the room’s ventilation system has a flow volume capacity of 236 L/s, it will take 8.7 minutes to reduce the acetone concentration to the required level, because:
Dt = |
V |
In |
Cinitial |
Q |
Cending |
Dt = |
1 650 |
In |
10 702 |
= 8.772 |
500 |
750 |
The question frequently asked is what chemical to use in the ventilation and reentry calculations. The answer is chemicals with highest vapor pressure and highest ACGIHSTEL and order threshold limits. The few specific chemicals used for such calculation in industry are acetone, acetaldehyde, chlorobromomethane, and cyclohexane.
HVAC system design for equipment support areas, glass wash areas, sterilizer facilities, conference rooms, offices, and so on should be based on actual loads and conditions. The A/E should thoroughly review the Program of Requirements to understand the scope and magnitude of miscellaneous space.
Laboratory buildings shall be supplied with multiple, manifolded air-handling units (AHUs) such that upon failure of any major component related to an AHU, the remaining available HVAC air-handling equipment will provide 100 percent capacity. A parallel system design using two or more pieces of air-handling equipment that operate simultaneously to meet full load conditions is the preferred choice to ensure overall system air-handling reliability. each AHU and its related components should be capable of being totally isolated with the help of isolation dampers located upstream and downstream from the remaining operational units to accommodate routine maintenance and emergency repairs in the event of equipment failure.
The laboratory exhaust systems, where there is no mixture incompatibility, should be arranged with multiple manifolded fans designed to maintain 100 percent of exhaust design conditions at all times. The number of fans should be determined by the A/E to accommodate physical and capacity restraints. One of the fans should be provided as a backup for any other single fan. Upon the loss of flow through any one fan, the designated backup fan shall be energized to maintain a constant exhaust system flow. The fan designated as a backup should be automatically alternated among all system exhaust fans so that all motors and equipment experience approximately the same running time. Exhaust fan motors and drives must be located out of the airstream. Each fan should be fully isolated from the others to accommodate routine service while the overall system is operational.
Exhaust air from laboratory equipment such as fume hoods and biosafety cabinets directed to a general central laboratory exhaust main is preferably controlled through pressureindependent terminal units. Supply fans should be energized after exhaust fans are operational and exhaust flow is confirmed.
No positive pressurized segment of any laboratory exhaust system should be located in any occupied zones including mechanical rooms. Offices within the mechanical rooms are classified as occupied zones. The design should permit the installation of exhaust fans at the end of exhaust lines and as close as possible to the final point of discharge to avoid or minimize leakage to the space, particularly mechanical areas. The positive pressure segment of an exhaust system should be constructed per the SMACNA standard for 1 494 Pa water-gauge positive pressure. A leak test should be performed to verify the SMACNA allowable leakage rate as defined in the HVAC Systems Duct Design Standard, Third Edition, Chapter 5. All ductwork shall be SMACNA seal Class A.
All toilet and general-use exhaust should discharge through a separate exhaust system and will not tie into kitchen/coffee room exhaust. In buildings housing both laboratories and other types of space with distinct occupancy zones in which the lab areas are segregated from other types of space, a separate HVAC system for the laboratory area is mandated. The HVAC designer is required to obtain approval from the NIH Division of Safety regarding exhaust mixture compatibility to avoid cross-contamination upon system failure or equipment damage due to an incompatible mixture.
As a minimum, supply air for these areas should pass through a prefilter and filter on the upstream side with efficiencies of 30 percent and 95 percent respectively, based on ASHRAE Standard 52.1-92, atmospheric dust-spot test efficiency. Special areas may require greater filtration on both the supply and the exhaust sides. The requirements for additional exhaust filtration shall be coordinated with the NIH Division of Safety, Occupational Safety and Health Branch and Radiation Safety Branch, where specific hazardous program functions occur.
Ventilation of environmental rooms such as cold rooms should be addressed on design documents. Those rooms that serve as occupied functioning lab spaces should receive minimum outdoor air ventilation at the rates defined by ASHRAE Standard 62-1989. Environmental rooms used primarily for storage functions do not require ducted ventilation air.
Air systems will require humidification to meet space humidity requirements using central plant steam. Low-pressure, dry-steam, direct injection humidification to introduce clean steam supplied by vaporizing softened water in a steam-to-steam generator should be used for humidification for special areas such as transgenic animal housing, barrier housing, and special patient areas (bone marrow transplant etc.). Duct humidifiers should be located downstream of fans. Ductwork within the absorption range of the humidifier should be stainless steel with a drainage facility.
Each individual room should be balanced for the actual airflow requirements (the highest cooling load or makeup air/ventilation airflow requirement). The central supply and exhaust air system should be balanced for the total of individual airflow requirements in each room plus the allowable duct leak based upon the SMACNA duct construction manual. A diversity factor should be applied if a variable air volume system is used. The central supply and exhaust air system should be sized on the basis of the following procedures:
- List the individual room total air requirements accounting for hoods, sensible heat loads, and minimum air change rates.
- Eliminate from the list those laboratory modules that currently have fume hoods.
- Calculate the additional airflow that would be required if 1.2 m, vertical sash fume hoods were added to half the remaining laboratory modules. Assume that the hoods are added to the rooms with the lowest airflows.
- Add the allowable total system duct leakage to the total from the previous item to determine future expansion requirements.
- Size all AHU system components and duct mains to allow for future expansion.
- Include in the system design the required airflow for not only present conditions but also future expansion. In the design calculation, describe the modifications that would be required to achieve the future expansion requirements and the reasoning behind the system sizing, including the life-cycle cost considerations.
- As a minimum, select main supply and exhaust fan motors one size larger than the required motor watts, and size the fan a minimum of 20 percent greater than the required present airflow L/s.
- Size individual exhaust and supply branches for the greater of the present airflow requirement or a 250 mm equivalent duct diameter.
F.11.8 Air-Handling Systems for Animal Research Buildings: The air-handling system design should comply with the requirements described in the Guide for the Care and Use of Laboratory Animals, current edition. The animal facility’s HVAC system design should be based on 100 percent outdoor air and should automatically compensate for pressure variations due to filter loading. Animal research facility air will not be recirculated. The system should be outfitted with pressure-independent hot water terminal reheating devices and humidifiers and provide individual space temperatures and humidity control. Individual control must be provided for each holding room, treatment room, procedure room, and operating room. The HVAC system shall be designed to individually control and maintain the proper temperature, humidity, differential pressure, and outdoor air exchange rate at all times within the facility. Where it is possible and program permitting, the similar spaces may be grouped to provide a single temperature and humidity control on approval from the Project Officer. The HVAC system capacity should be based on the largest of the five main parameters specified below:
- Minimum ventilation requirements of outdoor air changes per hour throughout the animal use area based in accordance with chapter 21 of the latest ASHRAE Applications Handbook and NIH publication Ventilation Design Handbook on Animal Research Facilities Using Static Microisolators, Volumes I and II.
- ASHRAE Transactions Section 2000 Volume 106, Part 1, 859-866.
- The amount of fume hood and downdraft table exhaust required to meet actual program requirements if there are animal research laboratories and procedure rooms within the facility.
- The required space-cooling loads to meet environmental conditions specific to the type of animal. This is primarily a function of thermal transmission, solar loads, associated laboratory support equipment, and animal and lighting loads.
- Minimum ventilation requirements as required to support microenvironments in ventilated cage racks.
Flexibility in design for easy conversion of the animal room from static cage racks to microenvironmental ventilated cage rack systems or a combination of both in the future should be provided.
If microenvironments are employed for animal holding, the minimum ventilation requirement may be reduced to 10 outdoor air changes per hour plus the sum of the microenvironment airflow. System connections to microenvironments should be designed to maintain manufacturer-specified criteria. For ventilated rack designs, it is recommended that airflow for animal rooms be derived by adding the airflow required for the animal heat loads of a fully loaded ventilated rack design and adding this airflow required for the room heat loads (lights, people, equipment, etc.). This airflow will be compared with the recommended airflow given by the ventilated rack manufacturers, and the larger airflow will be selected. If the animal room is to be designed for either ventilated racks or static racks, then the ventilated rack air change rate could be superseded by the air change rate required to handle the full heat load of an animal room with static racks.
HVAC systems serving animal facilities should be designed with parallel heating, ventilating, and air-conditioning system arrangements and/or with standby equipment with the capability to ensure continuous operation during equipment failure and scheduled maintenance outages. Parallel operation using two or more pieces of equipment, which operate alternately to meet full load, is the preferred choice. Whenever parallel equipment is not possible, redundancy is required by means of standby equipment that handles the full load and operates when the primary system fails. Each AHU and its related components should be capable of being totally isolated from the remaining operational units to accommodate routine maintenance and emergency repairs.
The exhaust system should be designed for and utilize a multiple-fan exhaust arrangement. The number of fans should be determined by the A/E to accommodate physical and capacity restraints. One of the fans should be provided as a backup for any other single fan. Upon the loss of flow through any one fan, the designated backup fan should be energized to maintain a required exhaust system flow. The fan designated as a backup should be automatically alternated among all system exhaust fans so that all motors and equipment experience approximately the same running time. Exhaust fan motors and drive must be located out of the airstream. Each fan should be fully isolated from the others to accommodate routine service while the overall system is operational.
In buildings housing both laboratories and other types of space with distinct occupancy zones in which the animal areas are segregated from other types of space, a separate HVAC system for the animal areas is mandated. Both areas may use a common standby unit if the situation permits to save cost. All toilet and general-use exhaust shall discharge through a separate exhaust system. The HVAC designer is required to obtain approval from the NIH Division of Safety regarding exhaust mixture compatibility to avoid cross-contamination upon system failure or equipment damage due to an incompatible mixture.
As a minimum, supply air for these areas should pass through a prefilter and final filter on the upstream side of the AHU with efficiencies of 30 percent and 95 percent respectively, based on ASHRAE Standard 52.1-92, atmospheric dust-spot test efficiency. Special areas may require greater filtration on both the supply and the exhaust sides. The requirements for additional exhaust filtration should be coordinated with the NIH Division of Safety, Occupational Safety and Health Branch and Radiation Safety Branch, where specific hazardous program functions occur.
High-efficiency particulate air (HEPA) filtration of supply air may be required for barrier housing or animal-holding rooms housing immunosuppressed or transgenic populations or locations where populations are involved in chronic testing. This is best accomplished by providing HEPA filters in the cage racks housing these species of small animals.
Specialty areas such as operating rooms, recovery rooms, and so on may require higher filtration levels. The A/E should assess the filtration needs for each function in coordination with research personnel.
In animal-holding rooms, supply air must be introduced through high-volume ceiling diffusers and uniformly drawn across animal-housing areas to provide uniform mixing in the room. Where required, a provision should also be made for high exhaust to be activated for directly exhausted cages to maximize the facility flexibility. Care must be exercised to ensure that the system does not create drafts on the animals and that the airflow is uniform in nature. Terminal velocity of discharged air 600 mm from wall surfaces must be less than 0.25 m/s or, in critical areas, 0.15 m/s at head height. The designer must follow the procedures detailed in Ventilation Design Handbook on Animal Facility and Animal Facility Design published by the NIH and the ASHRAE Applications Handbook.
Humidity control is critical in animal areas. Higher relative humidity in winter is often required for primates and certain other animals as compared with laboratories. Lowpressure, dry-steam direct injection humidification to introduce clean steam supplied by vaporizing softened water in a steam-to-steam generator should be used for humidification for special animal housing areas, transgenic animal housing, autoclaves and sterilizers, and so on.
F.11.9 Air-Handling Systems for Administration Buildings: Air-handling systems for administrative, office, conference, and other general use facilities are similar in design. They frequently employ variable air volume with terminal zone- or room-heating units. These systems are a recirculating type with ventilation rates designed to meet the latest ASHRAE Standard 62-2001. Air-side dry-bulb economizers provide free cooling when ambient conditions permit.
Air-handling systems for administration buildings are best kept simple and zoned consistent with building use and occupancy schedules. Large conference or assembly areas with intermittent use should not be connected to units that supply routine office space. Airhandling systems found in these buildings may have the following features:
- Single supply and return fans without redundant components
- Night setback and morning warmup control modes
- Mixing plenums with minimum and maximum outdoor air dampers to accom-modate minimum ventilation and economizer operations
- 30 percent efficient prefilters and 60 percent efficient afterfilters
- Preheat coils required to support morning warmup functions
- Drawthrough chilled water coils
- Central AHU humidifiers only
- 750-1 000 Pa pressure duct distribution to terminal control devices
- Fully ducted return air system with building pressure-controlled relief devices
Computer or data-processing facilities are commonly found in administration buildings and require special consideration. HVAC systems for central computer rooms must meet the special requirements for lower temperatures, controlled humidity, and extremely high reliability. The suggestions listed below are excerpted from the ASHRAE Handbook for HVAC Systems and Equipment and they shall be taken as requirements unless the program dictates specific equipment requirements that need to be further considered. The typical computer room design conditions temperature setpoint and offset shall be 22 ± 1 °C; the RH setpoint and offset shall be 50 ± 5 percent; and filtration shall be 45 percent minimum based on ASHRAE’s dust-spot efficiency test. A sensible heat ratio, approximately 0.9 to 1.0, is common for computer room applications.
The air-handling apparatus should be independent of other systems in the building, although it may be desirable that systems be cross-connected, within or without the dataprocessing area, to provide backup. Redundant air-handling equipment should be evaluated. Ventilation air provided by the building system is acceptable. The refrigeration systems should be independent of other systems and should be capable of year-round operation. It may be desirable to cross-connect refrigeration equipment for backup, as suggested for air-handling systems. Fire protection for the air-conditioning system should be fully integrated with fire protection for the computer room and the building as a whole. Computer room systems are attractive candidates for heat-recovery systems because of their large, relatively steady, year-round loads.
Administration buildings traditionally have large glass areas with a large diversity of load based on exposure and occupancy. Careful consideration given to the number and placement of terminal control devices is required. Each unique room should have a separate point of control. Rooms that are similar in size, use, occupancy, and exposure may be combined on a single point of control.
Perimeter radiation should be considered where large glass areas exist and where furniture layouts place seating adjacent to perimeter walls. The controls of radiation should be fully coordinated with other terminal control units so that simultaneous heating and cooling do not occur.
Toilet rooms, janitor facilities, pantries, lunch rooms, copy rooms, and other miscellaneous spaces require exhaust to remove odors and heat from occupied areas. Toilet rooms and janitor closets should be connected to common exhaust systems and be designed to run continuously. Other exhaust may be connected to general exhaust systems that are controlled to operate when central air-handling equipment is operational.
F.11.10 Air-Handling Systems for the Clinical Center Complex: The Clinical Center Complex buildings are generally designed using recirculating-type HVAC systems with various percentages of required outdoor air. Air-handling systems may be of the constant or variable air volume type provided that minimum total occupied air change rates are maintained. Systems should automatically compensate for filter loading and pressure changes. Pressure-independent hot water terminal reheat devices should provide individual space temperature control. The HVAC system should be designed to maintain the proper temperature, humidity, differential pressure, outdoor air exchange rate, and acoustic criteria within the space. The HVAC system capacity shall be based on the largest of the following parameters:
- The amount of total supply air required to satisfy the specific outdoor air exchange rate for each of the various program functions.
- The required space-cooling load, which is primarily a function of thermal transmission, solar loads, associated medical equipment, occupant load, and lighting loads. At the NIH, a combined nominal equipment and lighting load density of 54 W/nm2 shall be used as a minimum in design of clinical patient trial areas, 22 W/nm2 for equipment and 32 W/nm2 for general areas.
HVAC system design for specialty areas such as intensive care units, surgery suites, radiology rooms, cystoscopy rooms, treatment spaces, and so on shall be based on actual loads and design conditions. The A/E should thoroughly review the program of requirements to understand the scope and magnitude of miscellaneous spaces.
Clinical Center Complex buildings should be supplied with multiple manifolded AHUs such that, upon failure of any major component related to an AHU, the remaining available HVAC air-handling equipment will provide 100 percent capacity. A parallel system design using two or more pieces of air-handling equipment that operate simultaneously to meet full-load conditions is the preferred choice to ensure overall system reliability. Each AHU and its related components should be capable of being totally isolated from the remaining operational units to accommodate routine maintenance and emergency repairs.
F.11.10.1 Critical Use Areas: Critical use areas such as operating and cystoscopy rooms should have dedicated air-handling systems that are backed up by the central units for redundancy. Each operating or cystoscopy room should have an individual temperature, humidity, and pressurization control.
Dedicated supply, return, and exhaust air systems serving critical use areas should automatically transfer to emergency power in the event of normal power failure. The supply air system should be single duct with unlined, pressure-independent, constant-volume hot water terminal reheat devices and low-velocity terminal ductwork.
The AHU serving critical use areas should have prefilters, filters, a heating coil, a cooling coil, and a central humidifier (to raise the humidity level to 40 percent RH). The final filters should be located on the discharge side of the supply fan and humidifier with a diffuser section in between to ensure the uniform distribution of airflow over the filter surface.
The supply air duct on the downstream side of the final filters should have air-tight access panels at each elbow and at 6 m intervals on straight duct runs for cleaning and inspection. The air distribution for each operating room should have perforated laminar flow panels positioned around the operating table to maintain a vertical low- velocity, laminar distribution. A minimum of two exhaust registers in each operating room should be located diagonally opposite and 177 mm above the finished floor. The exhaust air quantity should be at least 15 percent less than the supply air (or 47.2 L/s per door minimum) to maintain positive pressure between the operating room and the adjoining areas.
A terminal humidifier on the downstream side of the dedicated constant-volume reheat terminal unit serving the space should maintain the individual humidity for each operating room. Terminal HEPA filters should be considered for use downstream of terminal humidifiers for each operating room.
Each operating room should be furnished with temperature and humidity recorders to keep a continuous record of ambient conditions. In lieu of the chart records, temperature and humidity sensors can be used to record data at the Building Control Center.
Where deemed necessary by program requirements, other areas such as intensive care units, isolation suites, and invasive procedure rooms may also be served by critical use AHUs.
F.11.11 AHUs and Components: The type and construction quality of AHUs approved for use in NIH buildings are based on several factors, such as size, system features, building types, site restrictions, and so on. The project engineer must carefully review the project design criteria to establish the most cost-effective equipment that provides, throughout the system life, stable and continuous operation. Major unit components should not require replacement until the system life is realized. The following guidelines should be utilized in the design and specification of AHUs:
- Air-handling systems that are generally small in capacity (less than 9 440 L/s), utilize return air, and are not serving critical program functions may be factory-packaged, institutional-grade units.
- Large, central station AHUs (greater than 9 440 L/s) that are recirculating or use 100 percent outdoor air should be a custom-designed, factory-fabricated and -tested unit.
- Large, central station AHUs designed for installation in existing buildings where access is restricted or designed for new buildings where the construction phasing does not permit the installation of large factory-fabricated sections should be custom-designed, field-erected and tested units.
The Basis of Design report submitted by the A/E should define the type and quality of airhandling equipment proposed for use during design. The report should provide justification for equipment selection by the A/E. Factory-packaged, institutional-grade AHUs, when approved for use, should conform to the following criteria:
- Units should be of a modular design and have double-wall casing for all component sections.
- The unit’s coil capacity must be able to handle up to 100 percent outdoor air when required, without moisture carryover.
- All unit components must have large, full-height access doors to permit inspection, routine service, and cleaning.
- Unit casings should be pressure rated for the total system design operating pressure plus 25 percent.
- Fan sections, where possible, shall employ airfoil fans with a minimum AMCA Construction Class of II.
- Fan sections should be isolated from the remaining unit and the connecting duct system to control vibration.
- Solid fan shafts only will be considered.
- External fan motors are preferred, and in all cases bearing lubrication lines should be piped exterior to the casing wall.
- Fan volume may be controlled using either inlet vanes or variable frequency fan drives.
- Units shall be in a draw-through arrangement.
- Coil drain pans should be stainless steel and have a positive slope-to-drain connection.
- Factory filter/mixing boxes may be utilized only for low-outdoor-air units and where filtration is limited to 30 percent prefilters.
- Built-up filter/mixing sections utilizing high-quality low-leakage dampers and filter frames installed within insulated metal casings are preferred.
- Waterproof lights at each section of AHU are recommended.
Custom-designed, factory-fabricated AHUs should be based on A/E contract documents and built to specific dimensions indicated therein. The A/E shall lay out, in sufficient detail, the desired arrangement of each complete unit showing all required components, access doors, casing openings, service clearances, and overall dimensions. Layouts should include sections to define the overall height and vertical location of duct connections, dampers, louvers, and so on. The factory-fabricated unit should be capacity and pressure tested as a completed unit at the factory before shipment. Custom-designed units and related airhandling system components should conform to the following criteria:
- Units should be custom engineered and preassembled at the factory on a structural steel base. The units should be shipped as one piece if possible or in as few sections as possible. The number of field-casing joints should be reduced at all reasonable cost.
- Casings should be factory fabricated and double walled with structural, acoustical, and thermal performance certified by testing data. Casings generally have a solid exterior shell with a solid interior shell upstream of final filters and cooling coils. A solid interior shell occurs downstream of final filters and cooling coils.
- Casing access doors are required for both sides of heating/cooling coils, fans, filters, dampers, sound attenuators, heat recovery devices, humidifiers, and any other component requiring routine service. Access doors, where possible, should be man sized (600 x 1 829 mm), have vision panels, and seal with the air pressure.
- AHU component sections should be supplied with suitable vapor-tight lighting to permit maintenance functions. Lights are typically controlled from a pilot switch located adjacent to the access door.
- Unit louvers should be AMCA rated and selected for low-pressure drop with less than 0.003 kg/m2 penetration at 3.8 m/s free-area velocity. Areaways for louvers should have a minimum of two drainage points sized for full capacity. Areaway floors should be sloped a minimum 8 percent to drain.
- Dampers should be low leakage and opposed or parallel blade as required, accommodating mixing of airstream. Opposed blade dampers are required for nonmixing applications. Particular attention should be given to achieve good mixing of outdoor and return air to minimize stratification and freezing of water coils. Air blenders should be considered for use when airflow arrangements do not support the effective mixing of different airstreams.
- Air filters may consist of cartridge-type elements; roll filters are not acceptable. Their design face velocity should not exceed 2.5 m/s nor should manufacturers’ standard nominal ratings be exceeded. The preferred filter face section dimensions are 600 x 600 mm. Outdoor air and return air as applicable should pass through prefilters. All filter banks should have intermediate supports to prevent bank deflection at maximum design pressure differentials.
- Minimum 30 percent efficient filters should be installed upstream of any heat recovery device.
- Preheat coils may be propylene glycol or hot water, steam, or steam with an integral face and bypass damper. All coils should have copper tubes with aluminum fins and galvanized casing. Steam coils should be drainable with vertical tubes and vacuum breakers. Glycol coils are generally preferred over hot water coils for added freeze protection. Hot water coils should have duplex coil-circulating pumps on a return line with automatic lead-lag control isolation check valves and provide 100 percent emergency power in the event of a pump failure. An individual starter should be provided for each pump.
- Cooling coil velocity should not exceed 2.5 m/s at maximum future and present design conditions. For new buildings, a coil shall be sized for a nominal face velocity not to exceed 2.0 m/s so that future growth can occur. Coils should have copper tubes with aluminum fins and stainless steel casing. Intermediate stainless steel drain pans should be provided for each coil bank more than one coil high. The cooling coil section should have a stainless steel drain pan and a positive slope-to-drain bottom connection. Pan drains should be properly trapped. For draw-through units where the drain pan is on the fan side, to counter the negative pressure on the trap relative to the outside, the trap height must account for the static pressure in the reverse direction. Where blow-over units are approved, the drain pan on the discharge side of the fan must have a sufficient trap height to account for the static pressure in the unit. Static pressure conditions accounting for the dirty filter must be used to calculate the trap height.
- AHU fans may be vane-axial, airfoil centrifugal (single or double width) or plenum fans as justified by life-cycle costing. Fans should have a minimum AMCA Construction Class of II. Fans should be totally isolated from the unit using inertia base and spring isolation. Fan volume control may be achieved using controllable pitch vanes on axial fans and either inlet vanes or variable frequency drives on centrifugal and plenum fans. Discharge dampers are not suitable for volume control. Fans shall be arranged in the draw-through position. Redundant or parallel fans should be installed in separate compartments and be capable of complete isolation.
- Where possible, sound attenuators should be integrated as a part of the AHU. The large cross-sectional area of most units results in low attenuator velocity and a corresponding pressure drop while maximizing the attenuator’s performance. The silencer rating should be determined in a duct-to-reverberant-room test facility, which provides airflow in both directions through the test silencer in accordance with ASTM E477.
- Custom units must be designed to be totally isolated from other adjacent units so that routine maintenance can occur with the unit off and other units operational. Ultra-low leakage, industrial-quality isolation dampers should be installed at the discharge of manifold units.
- Each AHU section should be provided with drainage facilities that permit the washdown of units and contain leaks resulting from coil failures.
- Casings should be constructed in a water- and air-tight manner. The manufacturer’s
standard cabinet construction should result in the latest ASHRAE/ANSI Standard 111 leakage class of less than 9 for demount units as measured in accordance with AMCA Standard Z10-85. The fully assembled unit should have a maximum air leakage rate of 1 percent of the supply air volume.
- Custom-designed, field-erected AHUs should be similar in many respects to those that are factory fabricated. These units basically arrive at the job site as individual components that must be assembled on concrete pads or curbs to form the unit. Casing construction quality and erection procedures are extremely important on these units. Poor-quality casings result in excessive AHU leakage and poor system performance. Contractor-shop-fabricated casings or filter frames are prohibited.
- When heat recovery equipment is used, the heating and cooling coils should be designed to function at full load with or without energy recovery. All coil schedules should show both entering and leaving air and glycol/water conditions. Units with heat recovery systems should be designed such that devices could be out of commission without any interruption to AHU system operation.
Humidifiers for central station AHUs should be of the dry-steam, manifold-jacketed or panel steam humidifiers (atomizing steam humidifier) type and be located in the duct system where possible. Ductwork within the absorption range of the humidifier should be at minimum 2 m upstream and downstream fully welded stainless steel and pitched to drain. Steam lines serving humidifiers should have an automatic isolation valve and be dripped to remove condensate prior to manifold. The isolation valve should be closed during cooling mode to prevent additional heat gain in the duct system. A high-limit humidity controller must be provided for each humidifier.
The installation of heating and cooling coils in AHUs often creates long-term maintenance problems. Coils installed in either factory-packaged or custom-designed units, if not properly engineered, will not be serviceable and will eventually fail to perform. The following issues shall be specifically addressed for all coil installations:
- Individual coils must be fully accessible on both the upstream and downstream sides to permit inspection and cleaning.
- The cooling-coil face velocity must be limited to 2.5 m/s across the entire face area to prevent carryover at maximum future and present design conditions. Air distribution plates may be considered for use upstream of coils, but plates induce a high pressure drop and should be avoided where possible.
- Moisture eliminators may be considered where carryover presents a problem; however, eliminators must not impede service access to the coil surface for cleaning.
- Multiple coils are often required to provide the total capacity of individual units. Coils shall be a maximum of 3.0 m long by 0.91 m high and be capable of replacement without major rigging. Individual coils must be removable without disturbing pipe headers or other coils.
- Multiple coils should be valved separately so that, if any individual coil fails, it can be isolated and drained while the remaining coils stay in operation. Return header for multiple-stacked coils should be piped reverse return to assist a balanced water flow at all load conditions.
- All coils should have integral vent and drainage ports. Steam coils should be nonfreezing vertical tube where installation is possible and provided with steam vacuum breakers, not check valves located outside the airstream. Condensate should not be lifted downstream of steam coils. Condensate lines should not be designed to discharge under pressure. There should be a hydraulic head between the coil and steam trap of 450 mm minimum.
- Even and consistent airflow across the entire coil surface is extremely important. Upstream mixing and the use of air blenders should be carefully considered.
- Coil bank supply and return mains or steam and condensate mains should have manual isolation valves so that the entire unit can be drained.
- Trainers should be provided on the feed line for each coil bank. Control and balancing valves should be installed on the return line for water coils. Balancing valves should be specifically designed for balancing and have integral memory stops. Combination balancing, shutoff, and flow meter devices are not acceptable.
- One-third and two-thirds steam control valve arrangements with a manual bypass valve should be considered for large steam coils to improve control and operating efficiency. Steam mains should be dripped prior to control valves.
- Float and thermostatic traps should be used on steam coils. Trap bypass lines should not be used; dual traps may be considered.
- Factory-packaged units should have offset coil pipe headers to allow individual coils to slide out of unit casings.
- Face and bypass coil arrangements used for temperature control in AHUs have not performed well at the NIH, nor have they provided the level of performance needed for proper operation. Integral face and bypass steam coils are preferred over standard face and bypass coils.
- Hot water preheat coils should be designed for parallel flow-circuiting. The counter flowcircuiting,
particularly with long coils, is dangerous because the cold air is in contact with the coldest water. Hot water flow should be maintained through the unit by a pump system in conjunction with use of a three-way control valve and a minimum of 40 percent glycol mixture as an antifreeze solution during freezing weather.
The A/E should give careful consideration to the location of the supply air fan with respect to coil banks. Excessive air velocity stratification across the face of a coil may affect the capacity, pressure drop, and water carryover characteristics. Thus, the location of the fan with respect to the coil bank is very important. Generally, if the air velocity across the coil does not vary by more than ±10 percent of nominal, essentially full capacity will be achieved and water carryover will not be a problem. However, if the air velocity stratification is greater than this, capacity reduction, carryover, and freeze-up problems could occur. When space limitations dictate that the fans be placed in close proximity to the heating or cooling coils, the following criteria should be used to determine the minimum distance between fan and coil for field built-up systems:
Draw-Through System: For single-width fans, the distance between the fan intake and coil should be a minimum of one wheel diameter. For double-width fans, the distance between the fan intake and coil should be a minimum of one-half wheel diameter.
Blow-Through System: Most problems occur in this type of system. To minimize space requirements, it is desirable to place the coil as close to the fan as possible. The minimum distance for satisfactory operation is a function of the dimensional relationship of fan to coil, fan outlet velocity, coil face velocity, and coil pressure drop.
When the coil must be located closer to the fan, baffles can be added on the face of the coil to increase their pressure drop to the required valve. Another possibility, which is usually reserved for field fixes, involves the installation of a baffle plate. A baffle plate with 50 percent free area should be placed two-thirds of the distance from the fan discharge to the coil. This baffle plate should have an overall area equal to four times the area of the fan outlet and be approximately the same shape. The pressure loss across this baffle will vary, of course, depending on the distance between the fan discharge and coil and the fan outlet velocity.
The contract documents should specifically address the placement of the fan with respect to the coil. Whenever possible, the fan should be placed with the outside of the fan scroll at or near the top or bottom of a coil bank.
F.11.11.1 Fans:Fans, both supply and exhaust, serving multiple zones, shall be equipment with either a VFD or a vortex damper or both for control of volumetric flow rate and duct static pressure. This is required for both variable and constant volume systems.
Fan systems designed for parallel or manifold operation shall have isolation dampers to prevent backward rotation of fan wheel. Isolation dampers shall have motors and shall be controlled by the BAS.
All fans on a manifold or in parallel shall be identical and have identical isolation dampers and volume/pressure controls.
All fans shall be fully accessible for service and routine maintenance. Fan motors and drives shall not be located within hazardous or contaminated exhaust airstreams. Fan bearings where possible shall be serviceable outside hazardous or contaminated exhaust airstreams. Inline fans with motors or drive exposed to exhaust airstreams are not permitted.
Fans shall have a certified sound and air rating based on tests performed in accordance with AMCA Bulletins 210, 211A, and 300. See AMCA Standard 99, Standard Handbook, for definitions of fan terminology. The arrangement, size, class, and capacity of all fans shall be scheduled on the contract drawings for permanent records.
Certified fan curves including power curves as well as acoustical data shall be submitted for each fan. All data shall be from test done in accordance with applicable AMCA standards.Data shall include published sound power levels based on actual tests on the fan sizes being furnished and shall define sound power levels (PWL) (10-12 W for each of the eight frequency bands).
Fan curves shall show volumetric flow rate of the fan as a function of total pressure, system flow rate as a function of total pressure, brake horsepower and efficiency. System curves shall include estimate losses for field installation conditions, system effect, and actual installed drive components. All losses shall be defined on the fan curves. Data may also be submitted in tabular form, but tables are not a substitute for actual performance curves.
All fans shall be statically and dynamically balanced by the manufacturer and shall be provided with vibration isolation. All fans 18.7 kW and larger shall also be dynamically balanced in the field by the manufacturer after the installation is complete.
All parts of fans shall be protected against corrosion prior to operation of the fan. Exhaust fans shall be specifically addressed, as the airstream may contain excessive moisture, fumes, corrosive vapors, or contaminated or hazardous particles. Special consideration shall be given to fans handling explosive vapors or radioactive material.
Each fan shall be equipped with a V-belt drive, except those that are direct drive by design. Belts shall be constructed of endless reinforced cords of long staple cotton, nylon, rayon, or other suitable textile fibers imbedded in rubber.
Variable-pitch sheaves shall be used to accommodate initial balancing and shall be replaced with fixed pitch when balancing is complete.
Sheaves shall be constructed of cast iron or steel, bored to fit properly on the shafts, and secured with keyways of proper size (no setscrews) except that for sheaves having 15 mm or smaller bores setscrews may be used.
Fans shall be furnished complete as a package with motors, drives, curves, bases, and inlet and outlet fittings.
F.11.11.2 Duct Design and Components: The duct system design for NIH buildings should consider space availability, space air diffusion, noise levels, duct leakage, duct heat gains and losses, balancing methods, fire and smoke control, initial investment cost, and system operating cost.
Deficiencies in duct design result in systems that operate incorrectly or are expensive to own and operate. Poor air distribution can cause discomfort; lack of sound attenuation may permit objectionable noise levels; and poorly designed sections of ductwork can result in an unbalanced system; faulty duct construction or lack of duct sealing produces inadequate airflow rates at the terminals; and insufficient duct insulation leads to excessive heat gain or loss and contributes to condensation problems.
The duct system design should be based on ASHRAE and SMACNA standards. Duct construction should be suitable for the operating parameters of the system and be tested to prove compliance with project specifications.
Fans in the field typically show a lower performance capacity than manufacturers’ ratings. The most common causes of deficient performance of the fan/system combination are improper outlet connections, non-uniform inlet flow, and swirl at the fan inlet. These conditions alter the aerodynamic characteristics of the fan so that its full flow potential is not realized. One bad connection can reduce fan performance far below its rating. The project engineer must consider potential field conditions and performance penalties in the final selection of fans.
Normally, a fan is tested with open inlets and a section of straight duct attached to the outlet. This setup results in uniform flow into the fan and efficient static pressure recovery on the fan outlet. If good inlet and outlet conditions are not provided in the actual installation, the performance of the fan suffers. To select and apply the fan properly, these effects must be considered, and the pressure requirements of the fan, as calculated by standard duct design procedures, must be increased.
To achieve rated fan performance, air must enter the fan uniformly over the inlet area in an axial direction without prerotation. Non-uniform flow into the inlet is the most common cause of reduced fan performance. Such inlet conditions are not equivalent to a simple increase in the system resistance; therefore, they cannot be treated as a percentage decrease in the flow and pressure from the fan. A poor inlet condition results in an entirely new fan performance. Many poor inlet conditions affect the fan more at near-free delivery conditions than at peak pressure, so there is a continually varying difference between these two points. The engineer must provide adequate space so that fan layouts can accommodate ideal inlet conditions. Poor fan layouts result in increased operating cost and deficient performance.
Since duct systems can convey smoke, hot gases, and fire from one area to another and can accelerate fire within the system, fire protection is an essential part of air-conditioning and ventilation system design. NIH guidelines and life safety codes require compliance with NFPA Standards 90A and 90B, which examine fire safety requirements for ducts, connectors, and appurtenances; plenums and corridors; air outlets, air inlets, and fresh air intakes; air filters; fans; electric wiring and equipment; air cooling and heating equipment; building construction, including protection of penetrations; and controls, including smoke control.
Leakage in all unsealed ducts varies considerably with the fabricating machinery used, the methods for assembly, and installation workmanship. For sealed ducts, a wide variety of sealing methods and products exist. Each has a relatively short shelf life, and no documented research has identified the in-service aging characteristics of sealant applications. Many sealants contain volatile solvents that evaporate and introduce shrinkage and curing as factors. Surface cleanliness and sealant application in relation to air pressure direction (infiltration and exfiltration) are other variables. With the exception of pressuresensitive adhesive tapes, no standard tests exist to evaluate the performance and grade of sealing products. Project specifications and ductwork plans should define the duct construction method and seal class A, sealing materials, and acceptable leakage rates for each application. Duct leakage tests shall be done in order to confirm construction quality and actual leakage rates.
Duct system design and air device selection and layout must consider the architectural aspects of the building. Ductwork must fit within the allocated space and not require the lowering of ceilings. Duct design must allow for easy adjustment and maintenance of required components. Air device locations must be coordinated with architectural reflected ceiling plan, bulkheads, lighting coves, and other special features. Air distribution systems are an integral part of the building and must be designed to meet the stated design criteria efficiently without generating noise, creating drafts, or causing thermal imbalances or poor IAQ.
Supply, return, exhaust, and outside air should be ducted for all spaces, i.e., not taken through ceiling plenums, shafts, mechanical equipment rooms, corridors, or furred spaces. Generally, the circulation of air directly between areas is not permitted, except into toilet rooms, locker rooms, and janitor’s closets. Circulation may also occur between adjacent corridors into a negative pressure area or out of positive pressure areas. Makeup air for kitchens or other food preparation areas may come from adjacent dining areas, since these areas are usually negative with respect to adjacent areas.
Conditioned air should be supplied to corridors to maintain design temperatures and as required to make up exhaust through negatively pressurized rooms opening directly to the corridor. The quantity of conditioned air to the corridors should be sufficient to maintain an overall positive building pressure. The A/E should develop a pressurization diagram as part of construction documents.
The supply air distribution system must be designed to minimize turbulence and to avoid impacting the performance of primary containment equipment such as chemical fume hoods and biological safety cabinets. Therefore, perforated ceiling panels located away from the containment devices are recommended to provide even and low terminal velocity performance instead of grilles, registers, and ceiling diffusers. If ceiling diffusers are used, the device should be placed away from the front of the hood, the quadrant of the device that blows at the hood face should be blocked, and the throw velocity of the device should be designed for no more than one-half to two-thirds of the hood face velocity.
Air distribution devices should be selected for each specific application. Many different types and styles of air devices are available in the marketplace to meet the various performance criteria. Discharge velocity, diffusion pattern, throw, terminal velocity, volume control, noise generation, and appearance are factors to be considered in device selection.
Air devices should be selected to provide a uniform, quiet, and low-velocity distribution covering the majority of the occupied area. Air devices should not dump the air, create drafts, or generate turbulence within rooms. Certain areas may require laminar flow devices to keep contaminants controlled below work areas until they are exhausted. ASHRAE’s HVAC Systems and Equipment Handbook, Air-Diffusing Equipment chapter, reviews the general application of the various air devices.
The terminal velocity of discharged air 0.61 m from wall surfaces desirably should be less than 0.25 m/s. Where applications become more critical, such as for laboratories, animal research facilities, and treatment/procedure rooms, the terminal velocity should not exceed 0.15 m/s at 1.8 m above floor height.
Table F.11.11.2.a summarizes the acceptable velocities for HVAC components and duct systems. Louvers require special treatment since the blade shapes, angles, and spacing cause significant variations in a louver-free area, pressure drop, and water penetration. Louver selections should always be based on data obtained in accordance with AMCA standards.
Table F.11.11.2.a Typical Design Velocities for HVAC Systems
Element |
Face Velocity (m/s) |
Ductwork |
|
Medium pressure mechanical rooms/shafts Occupied areas |
12.7 10.2 |
Low-pressure mechanical rooms/shafts Occupied areas Terminal outlets |
7.6 6.1 3.8 |
Outdoor/relief air |
7.6 |
Cooling/dehumidifying coils |
2.5 maximum |
Heating Coils |
|
Steam/hot water unit Ductwork Electrical |
2.5-3.8 7.6 maximum per mfg. data |
Filters |
|
Viscous impingement |
1.0-4.0 |
Dry-type, extended-surface Flat (low efficiency) |
duct velocity |
Pleated media |
2.5 maximum |
HEPA |
1.3 maximum |
Louvers |
|
Intake Exhaust |
2.5 maximum 3.8 maximum |
Ductwork may be either single- or double-wall construction as required to satisfy the acoustical requirements specified in these guidelines. Double-wall construction should consist of a perforated liner surface with an approved film-covering acoustical material. Terminal unit sound attenuators having a construction similar to double-wall ductwork may be utilized for room noise attenuation. The use of internal sound lining is prohibited at the NIH.
Ductwork may consist of either round, flat-oval, or rectangular shapes as needed to suit the building. Duct fittings, joint methods, supports, and construction details shall meet the requirements of SMACNA. All fittings should have documented flow loss coefficients by either SMACNA or ASHRAE. Irregular or makeshift fittings are not acceptable. Factory fabricated fittings by independent manufacturers may be utilized provided they have catalogued performance criteria.
Flexible ductwork may be utilized for supply air application to connect air devices to lowpressure duct mains and to make the final connection to terminal units. Flexible duct runs should be limited to 600 mm for terminal units and 1 800 mm for air devices. Flexible ducts shall have a UL-rated velocity of at least 20.3 m/s and a maximum UL-rated pressure of 2 490 Pa positive. Flexible ducts must be factory insulated and comply with the latest NFPA Standards 90A and 90B. Flexible duct joints should be made using stainless steel draw bands and manufacturer-approved tape.
The project engineer for each unique system installed on the project shall specify the duct construction method, material of construction, and pressure classification. Table F.11.11.2.b shows the minimum requirements for generalized applications in NIH buildings.
Table F.11.11.2.b Minimum Duct Construction Standards
Application |
SMACNA Pressure Classification |
Materials |
Low-pressure supply ductwork |
498 Pa POS |
galvanized steel |
Medium-pressure supply ductwork upstream of terminal units |
1 494 Pa POS |
galvanized steel |
Low-pressure supply ductwork downstream of terminal units |
498 Pa POS |
galvanized steel |
Low-pressure outdoor, relief, return air ductwork |
498 Pa POS |
galvanized steel |
Medium-pressure return duct work downstream of terminal units |
1 000 Pa NEG |
galvanized steel |
Low-pressure general exhaust ductwork |
498 Pa NEG |
galvanized steel |
Low-pressure wet process exhaust ductwork |
498 Pa NEG |
aluminum or stainless steel |
Low-pressure hazardous exhaust ductwork upstream of terminal unit |
498 Pa NEG |
epoxy-coated galvanized steel or stainless steel |
Medium-pressure hazardous exhaust ductwork downstream of terminal units |
Class I/industrial 1 494 Pa NEG |
epoxy-coated galvanized steel or stainless steel |
Special hazard exhaust ductwork |
1000 Pa NEG |
stainless steel |
Note: Galvanized steel duct may be used in lieu of epoxy-coated or stainless steel duct per IMC
recommendations if corrosion-inducing chemicals are not used in the fume hoods.
Duct leakage shall be tested and shall not exceed 3% of the total volume in low pressure systems or 0.5% in medium and high pressure systems. The duct leakage test shall be done as part of the required air balance by subtracting the sum of flows at all air devices from the total air flow measured at the air handler or exhaust fan. In addition the designer may require a leakage test to be done in accordance with the SMACNA HVAC Air Leakage Test Manual. If the SMACNA test is done, the test pressure shall be the design working pressure of the system.
Wet exhaust ducts or those duct systems that tend to carry moisture should be pitched toward the source of moisture generation. Drainage facilities should be provided in these systems.
The term “hazard exhaust” generally applies to common exhaust systems serving laboratories, fume hoods, animal research facilities, biosafety cabinets, and so on, which, by their relatively light hazard rating, may be exhausted by a common exhaust system serving BSL-2 areas.
The term “special hazard” generally applies to all other exhaust systems serving BSL-3, BSL-4, radioactive hoods, and so on, which, by their critical nature or extreme hazard, must be exhausted individually and normally require special filtration.
Wet-exhaust ductwork should be of either aluminum or Type 304 stainless steel construction to prevent corrosion. Hazardous exhaust or special exhaust ductwork should be at least Type 304 welded stainless steel or better as required to handle exhaust products.
F.11.12 Separation of Intakes and Exhaust: Outdoor air intake and exhaust discharges should be located to avoid health hazards, nuisance odors, reduction in capacity of airconditioning equipment, and corrosion of equipment caused by reentry of exhaust air from any source. The A/E should ensure that no cross-contamination will occur from exhaust discharges to outdoor air intakes.
Outdoor air intakes are classified as any louver, duct, gooseneck, ventilator, or grate pipe that is commonly used to take in outdoor air for the purpose of ventilation, heat removal, exhaust makeup, combustion air, air compressor makeup, or comfort conditioning. Exhaust discharge includes that from exhaust fans, vehicle exhaust, cooling towers, boiler or incinerator stacks, emergency generators, vacuum pumps, steam or other hot vents, plumbing vents, condensing units, kitchen hoods, relief from AHUs, and mechanical/electrical room ventilators.
Separating air intake and exhaust air outlets by at least 3.05 m as recommended by codes is a minimum requirement under normal conditions. Other factors such as wind direction, wind velocity, stack effect, system sizes, and height of building and security concerns must be evaluated, and location of intakes and outlets should be adjusted as required. The ASHRAE Fundamentals Handbook is a source for analyzing these factors. The facility manager should be consulted in the areas of physical security for placing air intake and exhaust. A common sense approach is required by the designer to evaluate the vulnerability of the HVAC system to a deliberate attack.
The bottom of all outdoor intakes should be located as high as practical but not less than 1.8 m above ground level, sloped or vertical not horizontal.
Use of an expert consultant to do either wind tunnel or computational fluid dynamics (CFD) air dispersion modeling is highly recommended to analyze and make recommendations on these factors. Where this is done, it must assess the possibility of re-entrainment of any and all near-by exhausts into any and all near-by intakes. For example, where a new building is being designed, the CFD or wind tunnel analysis considers the impact of the new building as well as near-by existing buildings and other new and
existing obstacles and considers new and existing exhaust relative to new and existing intakes.
When using CFD, certain factors should be considered in the evaluation of external flow type scenarios. First, the size of intakes, chimneys, and so on in an external flow problem in comparison to the overall size of the solution domain considered is usually small. In terms of creating computationally tractable problems, it is difficult to resolve the grid close to these sources of heat, momentum, or concentration without being subject to numerical diffusion. To highly minimize the numerical diffusion augments and the effective viscosity, the solution domain should use advance grids (meshing) or higher order differencing schemes. Second, the most widely accepted turbulence model used in CFD, namely the kturbulence model, over-predicts turbulent viscosity in regions of decelerating flow. Therefore, the model should be based on the assumption that the turbulent viscosity is the same in all three coordinate directions; that is, the viscosity is orthotropic. This is untrue for highly curved, swirling, or buoyant flows. All of these forms of flow regime are typically present in external flows of interest to some extent. The effects of this can be offset by alternatives, but they are subject to various problems.
To alleviate the concerns from the numerical simulation aspect, a series of grid refinement tests should be carried out to minimize the effect of numerical diffusion in this calculation. The numerical diffusion in three dimensions can be approximated, using Patankar (1980), as:

Where:
∆ = fluid cell density
V = fluid speed
(nx, ny, nz) = unit vector // to flow
dx, dy, dz = cell dimensions
Owing to the complex nature of this, the approach and the methodology of these calculations need to be agreed upon between the NIH and the contractor.
The results of such tests will be approved by the NIH. If it is found that the numerical diffusion issue cannot be addressed in a single model, then a “zoom-in” approach will be used. In this zoom-in approach, an initial model will be constructed that will represent the laboratory building plus all the surrounding buildings. The results from this initial simulation will then be taken from a volume immediately surrounding the laboratory building and applied to a second model that represents only the laboratory building and its immediate surroundings. If necessary, grid refinement tests will also be applied to the second model to ensure that numerical diffusion is eliminated as much as possible.
The following will be considered in this study. (Details and clear methodology for the calculations will be provided by the NIH. Contact Farhad Memarzadeh, Ph.D., P.E., ORF, for assistance and guidance.)
- A methodology for the calculation of reentrainment into the building.
- A methodology for the calculation of odor and health threshold limits (in mg/m3) and their comparison against the numerical analysis data.
- A methodology for the determination of pass/fail criteria based on the threshold limit.
- Alternate wind speeds and directions from appropriate wind rose data.
Outside air intake should be at least 12 m away from hot exhaust discharging horizontally or deflected down, plumbing vents, animal room exhaust, generator exhausts, loading docks, boiler flues, automobile entrances, driveways, passenger dropoffs, cooling towers, and incinerator and boiler stacks.
The A/E should use data, formulas, and other design information as published by ASHRAE, ANSI, and other sources in designing the exhaust stack height and velocity characteristics to overcome the building cavity boundary and avoid reentrainment of exhaust. Stacks shall be shown as part of the architectural design and the design rationale described in the early design submittal. In general, exhaust stacks should:
- Be in a vertical direction at a minimum of 3 m above the adjacent roofline and so located with respect to opening and air intakes to avoid reentry of contaminants into any building.
- Have a discharge velocity of at least 20 m/s.
- Be designed so that aesthetic considerations concerning external appearance are not allowed to overcome the above requirements and the safe discharge of exhaust.
- Be designed so that, where possible, multiple-manifold exhaust fans have separate exhaust stacks to avoid a positive pressure condition on the dischargeable side of an inoperable fan.
F.11.13 Exhaust Systems: NIH buildings typically contain a large variety of exhaust air systems that serve a multitude of program functions. The primary purpose of any exhaust system is to remove odors, vapors, hazardous materials, or other contaminants from buildings to protect the health and safety of building occupants. Exhaust discharge must be designed to prevent the reentrainment of exhaust back into outdoor air intakes.
F.11.14 General Exhaust Systems: The systems covered under this category are conventional, low-pressure, low-velocity types, serving toilets, locker facilities, janitor’s closets, soiled utility rooms, laundries, and trash rooms. The general exhaust system also includes areas with 100 percent exhaust requirements dictated by ventilation standards. The specific examples under this classification are central sterile supply areas and kitchens.
General exhaust systems are typically simple in design with control limited to start-stop modes as a function of occupancy schedule. Systems are low velocity without terminal control devices or redundant fans. General exhaust fans are typically supplied by normal power only. General room exhaust air should be exhausted through ceiling registers located over heat-producing equipment such as copiers, refrigeration equipment, appliances, computer equipment, and so on.
Wet exhaust from shower rooms and process areas is frequently handled by these systems. The moist air over sterilizers, glass/dishwashers, cagewashers, and pot-washing equipment should be captured using canopy-type stainless steel hoods. Exhaust air should be at a minimum rate of 254 L/s/m2 of hood area. Canopy hood duct connections should be fitted with moisture eliminators. Wet exhaust systems, where possible, should be separated from other general exhaust, and ductwork shall be either stainless steel or aluminum pitched toward inlets for drainage.
Air may be exhausted from the corridor through toilet rooms, bathrooms, and janitor’s closets if necessary. Exhaust air shall not be drawn directly from a public corridor through any storage room.
All kitchen or pantry hoods are furnished as dietetic equipment including the canopy hood over pot-washing stations. These hoods are furnished by the fabricators of the kitchen equipment and serve:
- Grease-producing equipment such as griddles, ovens, broilers, and deep-fat fryers
- Hot vapor-producing equipment such as steam kettles, vegetable steamers, and highpressure cookers
The exhaust systems serving these hoods have the following specific requirements. Filtered (30 percent efficient, Grade D) unheated and uncooled outside air shall be at the rate of 155 L/s per linear meter of slotted perimeter of the hood as the makeup air to meet the exhaust needs. Assume a 37 Pa water-gauge static pressure drop through the hood.
The remaining makeup air at the rate of 232 L/s per linear meter of slotted perimeter of the hood should be derived from the environmental air supplied by the kitchen and/or dining room AHUs.
Exhaust of 387 L/s per linear meter of slotted perimeter using a dedicated exhaust fan for each hood and 335.28 Pa static pressure drop through the hood should be assumed. See NFPA Standard 90A for the specific requirements of duct velocity, access, material, construction, and routing. Automatic operation should be provided for the exhaust fan through the hood control panel (furnished with the hood) for washdown cycle and fire protection.
F.11.15 Laboratory Exhaust System: The laboratory exhaust system, where there is no mixture incompatibility, should be arranged with multiple-manifolded fans designed to maintain 100 percent of exhaust design conditions at all times. The number of fans should be determined by the A/E to accommodate physical and capacity restraints. One of the fans should be provided as a backup for any other single fan. Upon the loss of flow through any one fan, the designated backup fan should be energized to maintain a constant exhaust system flow. The fan designated as a backup should be automatically alternated among all system exhaust fans so that all motors and equipment experience approximately the same running time. Exhaust fans should be of industrial grade with motor and drives located out of the airstream. Each fan should be fully isolated from the others to accommodate routine service while the overall system is operational.
The laboratory exhaust system should account for filter loading (only where filtration is a requirement) and will generally combine general laboratory and fume hood and biological safety cabinet exhaust for maximum optimization of space and to achieve better dilution levels with acceptable concentration rates. The system can generally be designed to exhaust laboratory air and all fume hood exhaust through the same duct system.
Special exhaust systems for fume hood exhausts that cannot be combined with the general laboratory exhaust, such as exhaust from perchloric acid fume hoods and radioisotope hoods, and so on, need to be identified early in the design process and incorporated into the design. Dedicated exhaust systems should be provided for those applications.
The HVAC designer is required to get approval from the NIH Division of Safety or the designated safety officer regarding exhaust compatibility to avoid cross-contamination upon system failure or equipment damage due to an incompatible mixture.
Exhaust air from laboratory equipment such as fume hoods and biosafety cabinets connected to a general central laboratory exhaust main is preferably controlled through pressure-independent terminal units. Exhaust fan systems used for fume hood exhaust from laboratories or specifically for dedicated fume hood exhaust shall be designed, selected, located, and maintained in full accordance with ASHRAE recommendations and SMACNA and NFPA standards. The exhaust fan system’s flow and pressure should be monitored by device and connected to the building automation system or otherwise to a visual alarm to alert Building Maintenance Office personnel upon loss of flow or pressure in the system. The alarm system should also indicate failure of any single fan in the system.
If filters or scrubbers are required, they should be located as close to the source of contamination as possible while maintaining ready access for installation, monitoring, and maintenance. Smoke or fire dampers shall NOT be installed in laboratory exhaust ducts serving fume hoods, biosafety cabinets, or other hazardous exhaust.
Supply fans should be energized after exhaust fans are operational and exhaust flow is confirmed. Fire detection and alarm systems should not be interlocked to automatically shut down laboratory hood exhaust fans except as formatted by NFPA Standard 45.
No positive pressurized segment of any laboratory exhaust system should be located in any occupied zones. Offices within the mechanical rooms are classified as occupied zones. The design should permit the installation of exhaust fans at the end of the exhaust line and as close as possible to the final point of discharge to avoid or minimize leakage to the space, particularly mechanical areas. The positive-pressure segment of exhaust system shall be constructed per SMACNA standard for 1 494 Pa positive pressure. A leak test shall be performed to verify the SMACNA-allowable leakage rate, as defined in the HVAC Duct Construction Standard, latest edition.
Fume hood exhaust ductwork and exhaust fans should be coated with a protective, corrosion-resistant coating or constructed of corrosion-resistant material, such as stainless steel or an epoxy phenolic coating, e.g., Plastite, Heresite, Keysite, or vinyl selected for resistance to the anticipated exposures. Galvanized steel duct in accordance with the International Mechanical Code may be allowed on approval from the Project Officer if it is established that there will be no corrosive chemical fumes present in duct exhaust.
Exhaust stacks should be located at the highest part of the facility and positioned to prevent reentrainment of fumes at intake points. Exhaust discharge must be at least 3 m above the adjacent roofline and located to prevent reentrainment into outdoor air intakes. Discharge of laboratory exhaust must be vertical with exhaust velocity of 18.3 to 20.3 m/s at the point of discharge. Reentry calculations shall dictate the specific exit velocity.
Vertical exhaust shafts should be combined in a plenum at the roof and exhausted with redundant exhaust fans in order to provide system reliability and to provide the ability to service one exhauster without system shutdown. Each exhaust fan should discharge separately above the roofline. Fan discharges should not be combined.
Fume hood air requirements will vary depending on the selected manufacturer’s requirement. Minimum face velocity of constant volume fume hood is 0.51 m/s across the opening of the fume hood at sash opened to 45-55 cm.
F.11.16 Animal Research Facility Exhaust System: Exhaust systems employed in animal research facilities are similar in use and function to those in laboratory buildings. General system requirements are identical. The system shall exhaust all hoods and animal research facilities air through a common manifolded system. Systems should be redundant, flexible, and reliable.
Exhaust air in general does not require filtration or scrubbing. However, exhaust as it leaves the animal rooms shall be filtered with a rough filter to capture hair and dander. A filter frame at low air exhaust that can hold a rough filter should be provided from animal housing rooms. In special cases involving infectious animals, a HEPA filter should be considered for use as directed by the NIH Division of Safety. These components should be designed and selected for durability and easy maintenance and replacement of filters.
Some special spaces in the animal research facility, such as BSL-3 treatment rooms or holding rooms, may require special filtration. Requirements for these special facilities must be determined with the users and the NIH Division of Safety on a specific-project basis.
Exhaust grilles should be located away from supply air diffusers in a manner that creates a uniform, low-velocity airflow across animal cages. Points of connections to the animal research facility exhaust system may be required for snorkels utilized to exhaust ventilated racks. The animal research facility exhaust system shall have a maximum air velocity of 7.5 m/s in exhaust ducts. The animal research facility exhaust system must be tied to emergency power so that ventilation continues in the event of a power failure.
F.11.17 Special Exhaust Systems: The special exhaust systems include dedicated exhaust systems for ethylene oxide (EtO) sterilizer, isolation rooms, autopsy suites, radioactive fume hoods, BSL-3 and BSL-4 facilities, and other miscellaneous functions as designated by the NIH safety officer. Each system has its unique set of requirements for air quantity, filtration, construction materials, type of discharge, controls, emergency power, hours of operation, and so on.
In general, each special exhaust system should have its own dedicated set of main and standby exhaust fan and ductwork not connected to any other exhaust system. To be in compliance with NFPA Standard 90A, exhaust ducts from the special exhaust systems should not be located in the same shaft carrying environmental supply and/or return air ducts.
A dedicated exhaust system should ventilate EtO sterilizer equipment, mechanical chase, battery-charging areas, floor drains, aeration units, and cylinder storage areas. The exhaust system should be separate from the general exhaust systems serving sterile processing areas. The exhaust systems should operate 24 hours per day and maintain negative pressure in the areas housing EtO equipment even when the supply air unit shuts down during unoccupied hours, weekends, and holidays.
- The discharge air from the exhaust fans should be released at the highest point above the building, and care should be taken to ensure that the discharge air does not shortcircuit and find a way into the intake of any AHU.
- The systems should be on standby emergency power.
The specific exhaust air intake locations listed above have different static pressure drops at the inlet. This information should be coordinated with the equipment manufacturer, and pressure-independent constant-volume terminal units in each branch duct, as required, should balance the system.
A dedicated exhaust system for isolation rooms should be capable of producing either a room negative pressure (normal isolation) or a room positive pressure (reverse isolation). A selector switch with an indicator should accomplish this mode. The isolation suite should have a dedicated exhaust system composed of a roof-mounted exhaust fan, bag-in/bag-out HEPA filter, and pressure-independent constant-volume terminal units and balancing devices. The system must be on standby emergency power.
The A/E should use data, formulas, and other design information as published by ASHRAE, ANSI, and other sources in designing the exhaust stack height and velocity characteristics to overcome the building cavity boundary and avoid reentrainment of exhaust into outdoor air intakes or adjacent buildings. Stacks should be shown as part of the architectural design and the design rationale described in the early design submittal. In general, exhaust stacks should:
- Be in a vertical direction at a minimum of 3 m above the adjacent roofline and so located with respect to openings and air intakes to avoid reentry of contaminants.
- Have a discharge velocity of at least 20.3 m/s.
- Be designed so that aesthetic considerations concerning external appearance are not allowed to overcome the above requirements.
- Concur in location and height with air dispersion modeling by the NIH Special Project Office.
- Exhaust so that discharge from individual fans are not combined but ducted independently through the roof.
A dedicated exhaust system for the autopsy/pathology suite should have the following specific features:
- The exhaust fan should be located at or near the roof with the fan discharging above the highest point of the building.
- A HEPA filter should be installed in the exhaust airstream near the source of contamination, where possible, to minimize contamination of ducts. For a short-run duct system a filter may be installed at or near the exhaust fan. Redundant bag-in/bag-out HEPA filter banks with isolation dampers shall accommodate maintenance of filters.
- A pressure-independent, constant-volume terminal unit should be provided in the exhaust airstream to maintain constant airflow with the varying system resistance.
- Two wall-mounted exhaust registers should be installed, one on each side of the sink and one above the top of the dissecting tables.
- Ceiling exhaust air registers should be located in the gross specimen storage room above the sink counter area to exhaust chemical fumes.
- Room air near each autopsy table should be exhausted by placing two wall registers approximately 175 mm above the finished floor.
- Approximately 24 L/s should be exhausted from the cold room (mortuary refrigerator) when the room light is on. An interlock of the exhaust damper with the cold room door switch shall be provided.
F.11.18 Machine Room Conditioning: Rooms covered by these guidelines include mechanical, electrical, elevator machine, boiler, autoclave, and cagewash equipment spaces. These spaces are typically not air-conditioned, but they are heated and ventilated to acceptable levels. Reasonable conditions must be maintained in these rooms for worker comfort, to increase equipment life, and to avoid excessive heat gains and losses to adjacent occupied areas.
Heating for equipment spaces generally consists of steam or hot water unit heaters sized to heat the space to 21 °C. Heaters should be strategically located so they can offset infiltration loads caused by leakage through louvers, ventilation rollup access doors, and so on. Isolated pipes within machine rooms do freeze and break during severe weather conditions and when other systems fail.
All machine rooms should be ventilated, as a minimum, to code requirements for maintaining acceptable IAQ. Virtually all rooms that house machinery will have internal heat gains that drive ventilation air quantities far above code minimums. Heat gains are generated by motors, transformers, heat exchangers, piping, tanks and vessels, dimmer banks, speed drives, and so on. The project engineer should itemize operating equipment and establish estimated heat gains for each space. Radiated heat gains from transformers, steam, and heating systems can be as much as 2 percent of demand loads. The ventilation systems should be sized using a 12 °C rise above ambient outdoor air conditions. This results in equipment rooms being as high as 40 °C in peak summer months.
Secondary switchgear rooms should have HVAC equipment to maintain 24-hour temperature between 18 and 26 °C and humidity maintained at 30 to 60 percent noncondensing to protect switchgear and electronic controls. Temperature and humidity sensors should be installed in switchgear/transformer rooms connected to the Supervisory Control and Data Acquisition (SCADA) system. An alarm printer in the high-voltage shop should be programmed to print high- and low-temperature alarms as well as dew-point alarms. This will allow shop personnel to respond to impending problems prior to damping temperatures and moisture.
For large equipment areas with significant air requirements, multiple fans are recommended so that ventilation can be staged by thermostatic control. When combustion equipment exists within a machine room, the ventilation requirements should be combined with combustion air load and the space maintained at a positive pressure. Combustion equipment requires forced ventilation whenever operating; therefore, freeze protection becomes a more critical issue in winter months. Combustion air is best handled using a heating and ventilating unit to temper the air before distributing to this type of room.
Elevator machine rooms, telecommunication closets, fire alarm rooms, and other similar spaces with electronic equipment may require air conditioning instead of ambient ventilation. The project engineer should define criteria for these spaces and design accordingly. Many times these rooms require air conditioning when building systems are off, thereby justifying the use of packaged spot coolers or fan coil units.
The National Elevator Code has specific requirements for ventilating elevator shafts and machine rooms that must be applied to NIH buildings. It is desirable to filter makeup air for ventilation of all machine rooms, but this becomes impractical because of large air quantities in many cases. Electrical room ventilation should always be filtered with efficiencies of 30 percent based on ASHRAE’s Standard 52, atmospheric dust-spot test efficiency. The National Electrical Code and National Elevator Code strictly prohibit the installation of mechanical systems in those rooms unless they serve the space. When locating heating equipment and routing piping within these areas, care should be taken to minimize the length of run and not to run over electrical equipment.
F.11.19 Emergency Generators: These guidelines serve as the basic criteria for the application of emergency power generators in NIH buildings. Virtually all buildings on the NIH campus use emergency power to back up life safety systems and supply critical equipment and spaces with power in the event of a normal power outage. Generator installations create numerous problems for the mechanical engineer that must be resolved in the early design stages. Requirements to be considered for installation are as follows:
- A location that is easily accessible for service and future replacement
- A mounting method to avoid structure-borne vibration
- An engine exhaust discharge location
- An engine-cooling system
- Ambient ventilation
- A fuel supply system
The following list of standards, issued by the NFPA, pertains to the installation and operation of generator sets. Installation standards and codes are subject to change and may vary by location or over time. The mechanical engineer has the responsibility for following all applicable local, State, and national codes and regulations.
- NFPA 30, Flammable and Combustible Liquids Code
- NFPA 37, Combustion Engines and Gas Turbines
- NFPA 54, National Fuel Gas Code
- NFPA 58, Liquefied Petroleum Gas Code
- NFPA 59A, Production, Storage, and Handling of Liquefied Natural Gas
- NFPA 70, National Electrical Code
- NFPA 99, Health Care Facilities
- NFPA 101, Life Safety Code
- NFPA 110, Emergency and Standby Power Systems
The generator location is determined mainly by related systems such as ventilation, wiring, fuel, and exhaust. The location should be away from high ambient temperatures and protect the generator set from adverse weather conditions and vandalism. The generator set should be close to the main power-consuming equipment.
The generator room must be large enough to include space for accessories such as batteries, control switchgear, transfer switches, and day tanks. Adequate access to the generator set for service and repair should be planned. At least 1.2 m of clearance should be provided around the generator set. There should be access for replacing the generator without moving the generator set or accessories, such as a day tank.
The location must be such that adequate ventilation can be provided to supply combustion air and remove heat dissipated by the engine, generator, accessories, and radiators. The location must allow the exhaust system to be routed to the outside. The exhaust system must be terminated at a location where engine exhaust will disperse away from buildings and building air intakes.
Generator sets located outside buildings must be protected from the weather. Integral weather protective housing is available for many models. When locating a set outside, the A/E should consider the risk of power disruption by wind, ice, snow, flooding, lightning, fire, and vandals.
The generator set should be located where engine, fan, and exhaust noise levels will be acceptable.
F.11.19.1 Exhaust System: The purpose of the exhaust system is to direct engine exhaust away from the engine and allow it to discharge into the atmosphere. A muffler should be connected into the exhaust system, either inside or outside the generator set enclosure. For maximum efficiency, and to prevent engine damage, the exhaust system should not create excessive backpressure on the engine. The correct pipe size, connections, and muffler should be selected to achieve proper operation of the generator. When exhaust piping runs through a floor, ceiling, attic, or concealed spaces, the exhaust pipes should be installed within a metal, masonry, or other approved chimney. The generator set exhaust system shall not be connected to an exhaust system serving other equipment. Soot, corrosive condensate, and high exhaust-gas temperatures will damage idle equipment served by a common exhaust system.
Generator sets that are installed outside a building with integral weather protective housing should have a mounted critical-grade muffler. The generator set should be located so engine exhaust will disperse away from buildings and building air intake and will not blacken walls and windows with soot. Every precaution must be taken to prevent excessive backpressure on the engine. Exhaust piping must comply with the following general safety precautions:
- Exhaust pipes should be steel and be strong enough to withstand the service. Schedule 40 black iron pipe is recommended.
- Exhaust pipes must be freestanding, not supported by the engine or muffler.
- Exhaust pipes must use vibration-proof flexible connector.
- Exhaust pipes must have a clearance to meet local and national codes for combustible materials and terminate outside the building.
- Exhaust pipes must be guarded to prevent contact with personnel, or severe burns could result.
- Exhaust pipes must be routed to avoid fire detection devices and automatic sprinkler alarm heads.
- Exhaust pipes must be vented to the atmosphere away from building doors, windows, and ventilation intake vents.
- Exhaust pipe routing and size must be designed to limit backpressure on the engine to within manufacturer’s tolerances.
- Exhaust pipes must be pitched downward and away from the generator set in a horizontal run or a condensate trap with a drain with hose connection, installed where a rise in the exhaust system begins.
- A flexible, corrugated stainless steel exhaust tube must be connected to the engine exhaust outlet to take up thermal expansion and generator set movement and vibration.
The mechanical engineer should select a muffler that will reduce the exhaust noise to an acceptable level. Three types of muffler are commercially available for the following applications:
- Industrial Muffler: suitable for industrial areas or remote installation where attenuation is not critical, 12 to 18 dB (A) sound reduction.
- Residential Muffler: suitable where some low background noise is always present, 18 to 25 dB (A) sound reduction.
- Critical Muffler: suitable for the areas of hospitals or residential dwellings or where background noise is minimal, 25 to 35 dB (A) sound reduction.
Residential or critical-grade mufflers are commonly used at the NIH depending on the location of the generator. The muffler should be installed as close as possible to the engine. Cool mufflers collect undesirable carbon residues and moisture. Draining and servicing the muffler are usually more convenient if it is installed near the engine. The muffler and exhaust piping should be insulated to prevent burns if accidental contact occurs and to reduce heat gains to adjacent spaces.
F.11.19.2 Engine Cooling: Liquid-cooled engines use a coolant that is pumped through passages in the engine cylinder block and heads, and sometimes through water jackets around the exhaust manifold. The coolant is pumped under pressure throughout the system. As the coolant moves through the engine, it absorbs heat from the engine. The coolant is then cooled by either a radiator or a liquid-to-liquid heat exchanger. The coolant consists of a solution of water and antifreeze suitable for the coldest ambient temperature expected.
Mechanical engineers may consider various methods of heat rejection including the use of factory-mounted radiators, factory-mounted heat exchangers, and remote cooling methods using various sources. The simplest method consisting of the factory-mounted radiator is the preferred choice of the NIH because it reduces maintenance and comes packaged with the generator.
Mounted radiators are installed on the bases of the generator set in front of the engine. A radiator-cooling fan draws air over the engine and pushes it through the radiator. This action provides surface cooling of the engine together with cooling of the engine coolant in the radiator. This method of cooling is independent of interruptible utility-supplied cooling water.
F.11.19.3 Ambient Ventilation: A room ventilation system is necessary to remove the heat and fumes dissipated by the engine, generator, accessories, and other equipment in the generator room. The system is also required to provide an adequate supply of clean combustion air. Ventilation system sizing should be based on the required air intake, maximum allowable total pressure drop, and type of engine-cooling system employed. Airinlet capacity must be sized to handle the combined flow of combustion and ventilation air.
Normally, there is an air inlet and discharge outlet in the room for circulation. Arrangement of these vents is such that air cannot escape without first passing through the immediate area of the generator set. Locating the outlet higher than the inlet allows for convection aircurrent flow. Ventilating air inlet and discharge openings must be located or shielded to minimize fan noise and the effects of wind on airflow. If louvers or screens inhibit free airflow, the vent areas should be increased by 25 to 50 percent.
Dampers or louvers protect the generator set and equipment room from the outside environment. Their operation of opening and closing should be controlled by the operation of the generator set(s). Dampers must be open when the set is running. Thermostatic shutters can be used to control airflow to maintain a desirable temperature range. They regulate airflow during operation and close at shutdown. Closing at shutdown is especially important in cold climates. Natural draining of cold air into the outlet duct can lower the ambient temperature below a safe level for all engines, especially diesels. In cooler climates, a movable or thermostatically controlled discharge damper can be used. This will recirculate radiator discharge air to keep the room warm when the generator set is operating.
The engine-driven fan set draws air forward over the generator and pushes it through the radiator. The radiator shroud has flanges for connecting a duct to carry the air to the outdoors. A flexible duct connector must be provided at the radiator to take up generator set movement and vibration.
Airflow through the radiator is usually sufficient for generator room ventilation. Auxiliary ventilation may be necessary if a low room air temperature rise has to be maintained. It is recommended that a maximum 12 °C room temperature rise be utilized in designing the ventilation air system. Certain generators may tolerate ambient conditions above 41 °C, but the maximum temperature should be limited to improve performance and generator reliability.
top
F.12 Fuel Supply
For continuous safe and satisfactory operation of emergency generators, an un-interrupted fuel supply system must be engineered and installed to industry standards. Generators may use either a liquid fuel or gaseous fuel source as justified by life-cycle costing. Liquid-fuel supply tank construction, location, installation, venting, piping, testing, and inspection must comply with applicable codes and NFPA Standards 30 and 37.
The supply tank must hold enough fuel to run the generator for the prescribed number of hours (NFPA 110 Class designation) without refueling. Tank-sizing calculations should be based on the hourly fuel consumption at full load. Other considerations for tank sizing include the duration of expected power outages versus the availability of fuel deliveries and the shelf life of the fuel. The shelf life for diesel fuel is 1.5 to 2 years.
For emergency power systems, codes might not permit the fuel to be used for any other purpose, or may specify a draw-down level for other equipment that guarantees the fuel supply for emergency power use. It is the NIH’s policy not to permit the generator fuel supply to be used for any other purpose.
For multiple-generator set installations, each set or its day tank should be connected independently to the fuel supply tank. This will prevent any set from starving for fuel when all sets are operating and prevent entrainment of air into the fuel system of idle sets.
Fuel tanks must be installed in accordance with code restrictions. The fuel tank should be as close as possible to the generator set. Because the fuel pump influences the fuel tank location, the fuel pump lift capability should be considered. If the sum of fuel pressure drop and vertical lift exceeds the lift capabilities of the standard fuel pump, the use of an auxiliary fuel pump and day tank is required.
Federal, State, and local codes have extensive requirements covering tanks and fuel piping installation. The A/E should carefully consider tank specialties, levelometer alarm and monitoring devices, and testing requirements.
When burying fuel lines, compatible metal fuel lines and fittings should be used to avoid electrolysis. Black steel pipe should be used for diesel fuel lines. Underground piping should be installed to protect it from contact with the building structure and include a leak detection and monitoring system.
A flexible section of code-approved tubing should be used between the engine and fuel supply line to withstand generator set vibration. Diesel-fueled generator sets require a fuel return line. All fuel line and tank fittings must be properly located and airtight to keep air from getting into the fuel lines. Fuel supply pipes and pumps must be sized to handle a flow rate three times greater than the full-load fuel consumption rate specified by the generator manufacturer. Fuel return pipes may be sized for twice the flow.
Elbows, bends, and long lateral distances in the fuel line reduce lifting capabilities. Note that in the descriptions of the various fuel systems using auxiliary fuel pumps, the vertical lift is limited by the capability of the transfer pump. With a large-capacity fuel pump, the vertical distance must not exceed 12 m lift. Fuel lifted long heights causes a pressure drop to the point where the fuel boils, produces a vapor, and causes vapor lock.
An electric solenoid shutoff valve in the supply line is always desirable and required for indoor automatic or remote starting installations. The solenoid wires are connected to the battery ignition circuit so the valve will open during operation.
Day tanks should be as close as practical to the generator set to provide direct fuel connections, at an elevation where the highest fuel level in the tank is lower than the diesel fuel injectors. Day tanks are fuel transfer tanks that are used when the standard engine fuel pump does not have the capacity to draw the fuel from the supply tank, or the supply tank is overhead and presents problems of high fuel-head pressure for the fuel return. The day tank is vented to the outside when installed indoors.
Diesel engine return-fuel should be piped to the supply tank rather than the day tank. Otherwise, the influx of warm return fuel will increase the day tank fuel temperature. As fuel temperature increases, fuel density and, consequently, engine power decrease. Return fuel should be piped to an intermediate day tank with float switch to pump fuel from there to the supply tank.
Gaseous-fuel supply system installations, operation, and maintenance must comply with all applicable codes and NFPA Standards 37, 54, and 58. For emergency power systems where the risk of interruption of offsite fuel supplies is high, codes might require an alternate onsite fuel supply and provisions for automatic transfer to the alternate fuel.
F.12.1 Types of Gaseous Fuels: The selection of a particular fuel depends on (1) availability, (2) efficiency required, (3) engine application (mobile or stationary), (4) initial cost, and (5) cost of operation.
F.12.1.1 Natural Gas: Natural gas is composed primarily of methane and varying amounts of other dry gases with a heat content of about 37.25 MJ/m3. It is piped from the source to points of consumption. Localities that are not serviced by natural gas will frequently have a manufactured gas system.
F.12.1.2 Manufactured Gas: Manufactured gas is not a particularly good fuel for generator sets if efficiency is important. Manufactured gas has a low heat value, and the engine will have to be de-rated as much as 50 percent. While gas manufacturing cost is usually higher than for other types of fuels, there are fewer storage problems and ambient temperatures have no effect on supplies.
F.12.1.3 LP: LP is a commercial mixture of propane and butane. The ratio between the two varies with local temperatures and user requirements. While propane vaporizes at a lower temperature than butane, butane has a higher heat content. Stored and transported under pressure in tanks, LP is a vapor at room temperature. By increasing pressure or lowering temperature, it remains in a liquid state. Liquid and vapor LP are both used as a fuel for generator sets.
F.12.2 Temperature and Pressure: Temperature and pressure are interdependent. If gas temperature is changed, the pressure will change proportionally. Gas at room temperature can be changed to a liquid by compressing and storing it in a closed container.
A liquid at atmospheric pressure can be changed to a gas by raising the temperature to the liquid’s boiling point. Vaporizing LP builds pressure within the container.
The fuel system components must operate at various working pressures depending on the kind of gas/vapor, size and length of fuel lines, number of generator sets supplied, ambient temperature, and so on. Components must have the strength to function properly under the anticipated or calculated maximum working pressures. LP tanks, for example, must have a minimum design pressure of 1 724 kPa per NFPA Standard 58.
top
F.13 Steam Systems
The steam requirements for NIH facilities are extensive and include autoclaves, cagewash equipment, kitchen equipment, HVAC systems, domestic water heating, and so on. Steam is generated at the NIH Central Heating Plant in Building 11 and distributed at a pressure of 1 138 kPa. Condensate is returned to Building 11 through a series of low-pressure pump return mains and high-pressure drip condensate piping.
Central plant steam is commonly used for most applications at the NIH. Clean steam produced from RO water or by double distillation should be used for humidification to special animal housing areas in animal research facilities, transgenic animal housing areas, special patient holding areas (bone marrow transplant etc.), autoclaves, and so on.
The type and method of steam/condensate distribution to NIH buildings should be thoroughly evaluated with life-cycle costing. The use of service tunnels, pipe trenches, and direct burial should be considered, and insulation alternatives should be optimized for energy savings. Where possible, all steam condensate should be collected and returned to the plant. Flash tanks should be utilized to reduce pressure, and the resultant flash steam utilized for low-pressure supply where a continuous demand exists. When the resultant flash steam has no established use, it should be vented to the atmosphere.
Steam and condensate distribution systems should be sized conservatively with minimal line pressure loss at maximum design load plus allowances for warmup and future growth. All valves, traps, equipment, and specialties should be selected and sized for their intended use by the project engineer. Sizing considerations should include warmup factors and estimated inlet and outlet pressure.
The room in which pressure-reducing valve (PRV) stations, condensate pumps, converters, and so on are located should be of suitable size to permit safe access for maintenance of equipment and safe, easy egress during emergencies. Main steam isolation valves should be automatic with manual bypass and located close to points of egress from mechanical rooms so that, in the event of a system failure, valves can be easily accessible for operation. Pump motor starters should be clearly identified and, where practical, shall be mounted on a common panel. If a duplex condensate pump is installed in the pit, the starter, disconnect switch, and alternator are to be located above the pit where easily accessible. Locating any serviceable equipment in a confined space should be avoided where possible.
Steam service rooms have excessive radiated heat gains from piping, valves, and receivers. All such equipment should be insulated to the fullest extent possible. Ventilation systems should maintain the room at 12 °C above ambient conditions or 40 °C.
The ASHRAE Handbook for HVAC Systems and Equipment and the ASME Code contain specific criteria for the design and installation of steam systems and should be utilized for NIH buildings, for which steam systems and distribution piping are classified as follows:
- Low-pressure steam: 97 kPa and below
- Medium-pressure steam: 103 kPa through 552 kPa
- High-pressure steam: 558 kPa and above
It is not uncommon in NIH buildings to have two medium-pressure steam systems (276 kPa and 552 kPa).
Steam should be supplied to the inlet of equipment steam control valves at the pressure indicated below:
- Radiators: 34 kPa maximum
- Convectors: 34 kPa maximum
- Air heating coils: 97 kPa maximum (higher pressures may be used if justified by engineering or economic considerations)
- Unit heaters: 207 kPa maximum
- Domestic hot water heaters: 552 kPa maximum
- Central humidifiers: 97 kPa maximum
- Terminal humidifiers: 97 kPa (duct mounted)
- Dietetic equipment: as specified by supplier
- Sterilizers and washers: as specified by supplier
- Heating water convectors: 552 kPa maximum
It should be noted that radiators, convectors, air-heating coils, and unit heaters are generally supplied with heating water in lieu of steam.
F.13.1 PRV Station: PRVs should be provided at the building steam service entrance or closest to user, as required to support building steam utilization. Two or three stations may be required and typically have the following reduction stages:
- High to medium: 1 138 kPa to 552 kPa
- Medium to medium: 552 kPa to 276 kPa
- Medium to low: 552 kPa to 97 kPa
Secondary or remote PRVs installed within the building are not desirable. Second-stage PRVs may be installed in mechanical penthouses or other easily accessible mechanical spaces. Small PRVs that serve isolated equipment such as glass washers with different pressure requirements may be installed close to that equipment in a service corridor or other suitable space. In no case should high-pressure steam be reduced in a single stage to either 276 kPa or 97 kPa.
PRV stations should be sized for the calculated peak demand of building heating, domestic hot water, humidification, and process equipment load. For process equipment load, use 100 percent steam consumption of the largest single user plus 25 percent steam consumption of all other users. Station sizing shall include provisions for future growth.
Where a single PRV would exceed 75 mm in size or the turndown ratio (maximum load/minimum load) is greater than 254 mm, two PRVs should be provided in parallel, one for approximately 0 to 33 percent for low-load conditions and one for 33 to 100 percent for high-load conditions, with a single full pipe size bypass. For large PRVs where valve sizes are exceeding 150 mm, three PRVs should be provided in parallel, one for approximately 0 to 33 percent for low-load and two for 33 to 100 percent for high-load conditions with a single bypass.
Where the steam service includes capacity for future expansion, all PRV station pipe and components except the PRVs should be sized for the future. The PRVs should be sized for the present load. Install eccentric reducers before PRV and a concentric reducer after the valve so condensate does not collect at the bottom of the reducer.
All PRV valves should be selected, and both the required load and the maximum capacity (for safety valve sizing) should be scheduled for that valve.
The PRV bypass valve and the safety valve should be sized according to the National Board Inspection Code of the National Board of Boiler and Pressure Vessel Inspectors (Columbus, Ohio). The safety valve should be sized to handle the maximum flow of the largest PRV or the bypass. The bypass valve capacity must not exceed the capacity of the safety valve. Bypass valves should have two isolation valves, the first valve to secure steam and the second to modulate pressure.
PRV stations and headers should be fabricated using fully welded fittings; flanged base plates are not acceptable. The high-pressure main should have a single shutoff valve capable of securing all steam to the building. Each branch of the PRV station shall have a single shutoff valve capable of securing steam without approaching the station.
PRV stations must be isolated from the structure to limit structure-borne noise. The maximum valve NC level should not exceed that specified at all anticipated loads. A noise suppressor should be provided as required. PRVs should be fitted with custom fabric insulation jackets to further reduce noises and heat gain to the space.
Steam valve pilot lines must be sloped down to tie into mains and must be contained within isolation shutoff valves. Pilot lines should be at least 15 mm to prevent clogging.
F.13.2 Condensate Return Units: Condensate receivers should serve only low-pressure mains and the discharge from flash tanks. Receivers should not be used as flash tanks or have high- or medium-pressure condensate directly piped, regardless of capacity. Condensate return units may be duplex electric, steam, or compressed-air powered. Electric pumps should be centrifugal, 26 rad/s maximum with Viton seals and stainless steel shafts. Each pump should have isolation valves on both the inlet and discharge lines to accommodate service.
Each condensate return unit should be piped with a full-size bypass line to drain. The bypass serves as emergency manual drainage for condensate if the return unit is off line. The bypass should be indirectly piped to the sanitary system and have a cooling trap to temper condensate down to a suitable temperature prior to discharge. All condensate receivers should be vented outdoors and independent of steam relief vents. Condensate return units should have fully packaged controls, starters, disconnects, and high-level alarms tied to the building automation system.
F.13.3 Steam Traps: Steam traps should be sized for their particular application. The safety factor to use in the selection will depend on the accuracy of the estimated load, estimated pressure at trap, and estimated backpressure. Safety factors based on type of trap and application of trap should be as follows in Tables F.13.3.a and F.13.3.b.
Table F.13.3.a Safety Factors for Steam Traps
Type of Steam Trap |
Safety Factor |
Balanced-pressure thermostatic traps |
2-4 |
Thermostatic traps |
1.5-2.5 |
Liquid expansion traps |
2-4 |
Bimetallic traps |
2-3 |
Float and thermostatic traps |
1.5-2.5 |
Inverted bucket traps |
2-3 |
Thermodynamic traps |
1.2-2 |
Table F.13.3.b Steam Trap Safety Factor
Application |
General |
With Temperature Control |
Mains, drainage |
x2 |
----- |
Storage heaters |
x2 |
----- |
Unit heaters |
x2 |
x3 |
Air-heating coils |
x2 |
x4 |
Submerged coils (low-level drain) |
x2 |
----- |
Submerged coils (siphon drain) |
x3 |
----- |
Rotating cylinders |
x3 |
----- |
Tracing lines |
x2 |
----- |
Platen presses |
x2 |
----- |
Rule of Thumb: Use a factor of 2 on everything except temperature-controlled air-heater coils, converters, and siphon applications.
F.13.3.1 Steam Trap Applications: Numerous types of steam traps are available, and each may serve a multitude of applications. Table F.13.3.1 lists various trapping applications and provides first and second recommendations for each case.
Table F.13.3.1 Recommendations for Steam Trap Applications
Application |
Float/Thermo-static |
Float/ Thermostatic With Steam Lock Release |
Float/ Steam Lock Release |
Thermo-dynamic |
Balanced Pressure Thermostatic |
Bimetallic |
Liquid Expansion |
Inverted Bucket |
Canteen Equipment |
|
|
|
|
|
|
|
|
Boiling pans, fixed |
A |
B |
B1 |
B1 |
B |
|
|
|
Boiling pans, tilting |
|
A |
B |
|
B |
|
|
|
Boiling pans, pedestal |
B |
B |
B1 |
|
A2 |
|
|
|
Steaming ovens |
|
|
|
|
A2 |
|
|
|
Hot plates |
B |
B |
B1 |
|
A2 |
|
|
|
Fuel Oil Heating |
|
|
|
|
|
|
|
|
Bulk oil storage tanks |
|
|
|
A |
|
|
|
B1 |
Line heaters |
A |
|
|
|
|
|
|
B1 |
Outflow heaters |
A |
|
|
|
|
|
|
B1 |
Tracer lines and jacketed pipes |
|
|
|
B |
A3 |
B |
B |
|
Hospital Equipment |
|
|
|
|
|
|
|
|
Autoclaves and sterilizers |
B |
B |
B1 |
|
A |
|
|
|
Space Heating Equipment |
|
|
|
|
|
|
|
|
Shell and tube heat exchangers |
A |
B |
B1 |
|
|
|
|
B1 |
Heating coils and unit heaters |
A |
B |
B1 |
|
|
|
|
B1 |
Radiant panels and strips |
A |
B |
B1 |
B1 |
|
|
|
B1 |
Radiators and convection cabinet heaters |
B |
|
|
|
A |
B |
|
|
Overhead pipe coils |
B |
|
|
|
A |
|
|
B |
Steam Mains |
|
|
|
|
|
|
|
|
Horizontal runs |
B |
|
|
A |
B2 |
|
|
B |
Separators |
A |
|
|
B |
B2 |
|
|
B |
Terminal ends |
B |
|
|
A1 |
B2 |
|
|
B1 |
Shutdown drain (frost protection) |
|
|
|
|
B3 |
|
A |
|
A = first choice for application
B = second choice for application
Refer to notes below for 1,2,3 suffix.
Notes:
1. Apply trap with air vent in parallel.
2. Install at end of cooling leg; minimum length, 0.91 m.
3. Use special tracing traps that offer fixed-temperature discharge option.
Reference: Steam trap data were taken from Spirax Sarco Application and Hook-up Data.
F.13.4 Piping Systems (Aboveground Steam and Condensate): Piping should be designated and installed to allow for expansion and contraction without creating excessive stresses and strain in the system. Expansion loops, offsets, and bonds should be provided as shown on contract documents and wherever possible. Expansion joints should be provided as a last resort. Pipe anchors should be designed for each location and sized to handle all forces with conservative safety factors. All anchors, guide loops, and joints must be readily accessible for maintenance and inspection.
Regardless of steam and condensate pressure classification, all pipe and fittings should be rated for minimum 136 kg at the NIH. Steam piping shall be a minimum Schedule 40 and condensate piping a minimum Schedule 80. Steam connections to equipment 50 mm and larger must be flanged and threaded for sizes 40 mm and smaller. Flange gaskets and bolts should be suitable for operating pressures and temperatures of the system. Hardware should be selected so that temperature and pressure fluctuations in the system and expansion/contraction do not affect performance over time.
Steam mains should be drip-trapped to accommodate condensate drainage at all locations. Drip connections should be provided at the base of each low point in mains and just before all equipment connections.
Condensate piping must be gravity drained after steam-consuming devices such as coils, heaters, sterilizers, and so on. There should be a hydraulic head between the trap and coil of 450 mm minimum to ensure drainage. Where the hydraulic head is not achievable, condensate pumps must be utilized. Under no circumstances should condensate be lifted after a modulating device, and condensate must drain freely by gravity.
High-pressure drip lines on steam distribution mains should be routed to individual buildings and not connected to pumped return lines to plant. High-pressure and medium-pressure condensate should be piped independently to an individual building’s flash tanks before connection to the condensate receiver. Flash tanks should be factory fabricated and ASME stamped and approved. Contractor shop-fabricated tanks are not acceptable.
A float and thermostatic (F&T) trap with leak sensor and check valve is the choice of preference at the NIH for low-pressure equipment. Traps must be sized for present load with a warmup factor. Condensate should not be lifted downstream of float traps. Trap bypass valves should not be installed; if redundancy is required or capacity dictates, dual traps should be installed. On approval from the NIH, thermodynamic steam traps for constant loads and F&T traps for intermittent loads may be used as required for the application to design the system.
All steam relief valves should be piped individually and discharged 2 100 mm above the building roof. Care should be taken not to locate discharges close to outdoor air intake or where they could be a hazard to maintenance personnel. Relief valves should not be connected to other steam vents. All valves, drip-pan elbows, and relief lines must meet ASME requirements.
Steam valves and specialties should be of the industrial high-performance type. Positive shutoff and isolation of mains are critical to the safety of maintenance personnel. Stainless steel seats and disk are required. Steam strainers should be positioned horizontally (flat) to prevent condensate from collecting in the bottom of the strainer and reducing its life.
Steam vacuum breakers, not check valves, should be used on coils and heat exchangers to eliminate vacuum. Vacuum breakers should be located external to air-handling unit casing. One-third/two-thirds control valves should be utilized for all heat exchangers and for coils where control is critical or capacity is large. Steam pressure gauges should be liquid filled with a range consistent with operating pressure. Stainless steel ball valves should be used for gauge cocks. Warmup valves 20 to 25 mm in size should be provided on each steam main depending on its size.
Steam to lab process equipment should have a drip leg installed before connection to prevent condensate buildup. Condensate must drain by gravity away from lab process equipment. Steam instrumentation sensors require a 600 mm-long sensing line from header to sensor to protect it from extensive heat.
Steam control valves should be fully proportional with modulating equal percentage plug. Steam valves should be designed to modulate and be sized to meet loads at full and partial loads. All steam control valves should have stainless steel trim and be suitable for the pressure condition and should operate with the differential pressure required. All steam valves shall be a minimum 1 725 kPa. All valve stems on systems to be insulated shall be provided as extended type as required to permit sufficient clearance for proper operation without damaging insulation.
Steam and condensate piping within NIH buildings should be sized for the parameters in Table F.13.4
Table F.13.4 Parameters for Steam and Condensate Piping
Steam Service |
Supply Mains and Risers Maximum |
Return Mains and Risers Maximum |
Drop kPa* (%) |
Friction Rate (kPa/30 m) |
Drop kPa* (%) |
Friction Rate (kPa/30 m) |
High-pressure system |
10 |
14-55 |
10 |
14 |
Medium-pressure system |
5 |
14 |
5 |
7 |
Low-pressure system |
5 |
|
5 |
|
* Percentage of supply or return main initial pressure.
top
F.14 Chilled-Water Systems
Chilled water should be provided from the existing NIH central chilled-water distribution system. The building chilled-water supply design temperature shall be 6 °C. The A/E should select the chilled-water heat transfer terminals to ensure that the interior space relative humidity is maintained at full- and part-load conditions with a leaving chilled-water temperature from the building at 16 °C.
Individual building (tertiary) chilled-water pumps shall be provided including 100 percent standby capacity. The system primary and secondary pumping is provided at the central plant. All terminal HVAC coils and equipment shall be provided with two-way control valves. The use of three-way control valves is prohibited. The selection of the terminal heat transfer equipment shall be made in conjunction with the control valve to maintain 16 °C leaving water temperature at all part-load conditions. Each of the building chilled-water pumps shall be variable speed. The speed of the pumps should be varied from differential pressure across a remote and representative portion of the building’s chilled-water system.
Special areas such as operating rooms and computer rooms may have dedicated chilledwater pumps installed with a hydraulic bridge. Dedicated or general-use chilled-water pumps serving critical areas should be provided with emergency power. Turbine or Venturitype water-flow measuring devices in main chilled-water piping shall be provided.
All valves and accessories should be arranged in a systematic manner in places accessible for maintenance and operation. All valve stems on systems to be insulated shall be provided as extended type as required to permit sufficient clearance for proper operation without damaging insulation. Access doors should be provided for valves in concealed spaces. Crossing of building construction joints with chilled-water pipes should be avoided. Chilledwater piping within buildings should be sized for a maximum velocity of 2.4 m/s and a unitary pressure drop of 1.2 m per 30 m of piping. Chilled-water piping from the distribution system to the building should be sized for a maximum velocity of 0.06 m/s and a unitary pressure drop of 1.2 m per 30 m of piping. For large facilities with floor areas greater than 9 290 gross m2, consideration shall be given to providing multiple building chilled-water services to the structure.
F.14.1 Secondary Chilled-Water/Process Cooling Water Systems: Secondary chilledwater systems used in NIH buildings serve fan coil units, refrigerant condensers, and other miscellaneous cooling equipment. The operating temperatures of these systems should closely follow that of the central chilled-water so that secondary equipment efficiency is optimized.
Secondary chilled water is most often generated by the central chilled water through plate and frame heat exchangers. Two heat exchangers of 100 percent capacity each must always be provided so that one can be serviced with the system operational. The central chilled-water system is operational year-round but is prone to occasional outages to accommodate maintenance functions and new construction. When the secondary chilledwater
system serves critical equipment that must remain in operation continuously, the A/E should consider the use of a supplemental building chiller to back up the central system. Supplemental chillers may be in direct contact with the secondary loop or be connected through three fluid-plate and frame heat exchangers.
F.14.2 Process Cooling Water: Process cooling water systems used in NIH buildings serve equipment or research functions that are not related to the building HVAC systems. These systems may have a highly diverse range of operating temperature and pressures that will eventually force the use of multiple systems. The A/E must define in the early design stages the design criteria for all such systems. The mechanical engineer should be an active participant in programming meetings. Process cooling water may be generated by the central chilled water of a building as condenser water source depending on the required operating temperatures. Plate and frame heat exchangers are commonly used to segregate the systems. Two heat exchangers of 100 percent capacity each must always be provided so that one can be serviced with the system operational.
Process cooling water systems are extremely critical because of the functions they serve. The system must have two reliable sources to generate the cooling water. When central chilled water is utilized, a supplemental chiller should be employed, and when condenser water is used, domestic water can be the backup. It may also be desirable to connect domestic water to the process side locally adjacent to the equipment for further reliability. Whenever domestic water is employed, it should be measured and charged to the Institutes so it is not arbitrarily wasted. Process equipment such as lasers may have very specific pressure limitations on both the inlet and discharge sides. Local pressure-reducing valves may be required to protect equipment. Other equipment may have extremely high pressure drops and require the use of local booster pumps. It is also not uncommon to have local 20 micron filters adjacent to equipment to improve the quality of cooling water before it enters the equipment.
F.14.3 System Design: The systems may be either constant or variable flow based on the control valve arrangement. Variable flow has proven to be highly effective because of the large diversity of loads and ever-changing pressure requirements. VSDs for each pump should be considered for improved reliability.
Each independent fluid connected to the systems should have its own expansion tank, air separator, and water makeup provisions. Supplemental chillers may operate in winter months and therefore will have ethylene glycol automatic fill equipment. The water quality in those systems is extremely important to reduce main-tenance and eliminate fouling in research equipment. High-efficiency strainers and local water filters should be integrated with the design.
Piping for these systems consists primarily of Type K copper for sizes 100 mm and smaller. Schedule 40 galvanized steel may be used only for sizes 125 mm and larger. Piping should be sized per the following criteria:
- Copper pipe: 1.2 m per 30 m maximum head loss and 1.8 m maximum pipe velocity
- Steel pipe: 1.2 m per 30 m maximum head loss and 2.4 m maximum pipe velocity
Piping for these systems is normally extensive and routed to the remote areas of all buildings. During the schematic design phase, the design engineer should develop a piping concept that reduces long, small-size terminal runs and accommodates easy system balancing to all equipment. Wherever possible, reverse return piping networks should be employed, as they tend to be self-balancing and force flow to the remote ends of the network.
Each piece of equipment should have a means to balance the water flow, determine the water flow, and control the capacity. Large-capacity equipment, over 0.63 L/s, should have pipe-mounted flow meter fittings. Smaller items should have pressure/ temperature plugs on the supply and return mains to accommodate balancing.
Dual primary distribution pumps are always base-mounted, end-suction or split-case, double-suction pumps. Close-coupled pumps are also acceptable on smaller applications. All pumps, coil circulating and distribution, should have the following components to support maintenance operations:
- Isolation valves on suction and discharge lines
- Pipe strainer or suction diffuser with strainer
- Flexible connections on suction and discharge
- Check valve on discharge
- Balancing valve with memory stop on discharge
- Pressure gauges and thermometer on suction and discharge lines
- Pump inertia base and vibration isolation when not installed slab on grade
- Pump speed not exceeding 1 750 rpm
- Discharge control valves where required for pump starting or equipment isolation
top
F.15 Hydronic Heating Systems
Hydronic heating systems are used for various services in NIH buildings. They may serve preheat coils, reheat coils, perimeter radiation, fan coil units, and domestic water heaters. The building size and design criteria often dictate whether a single system or multiple systems supply all services.
Regardless of the equipment served by the system, most hydronic heating systems have similar components and piping arrangements. A typical system consists of a shell and tube convector, duplex distribution pumps, expansion tank(s), water makeup provisions, air separator and two- or three-way terminal device control valves.
Hydronic heating systems normally have main and standby components with 100 percent capacity in the event that lead components fail. On large systems, three sets of convertors and pumps can be provided, two to carry the load and one as standby.
Each shell and tube convertor is supplied with either low- or medium-pressure steam and provided with one-third and two-thirds capacity control valves for uniform temperature control. Single control valves may be utilized for low-capacity convectors and where close temperature control is not required. Steam mains must always be dripped adjacent to converter connections to ensure a good-quality steam flow to the convertors. Condensate discharge should be lifted downstream of convertors unless it is pumped. Single condensate traps are normally provided for each converter without bypass piping. Where large convertors are installed, the A/E may design duplex traps of 50 percent capacity each.
The entire hydronic heating system may be provided in a manufactured package depending on its size. Packaged systems are typically skid mounted, pre-piped, and pre-wired. The manufactured package must be of sufficient size to accommodate service of all components and replacement of major parts without shutting off the entire system and should allow easy maintenance and access to all components.
The systems may be either constant or variable flow based on the control valve arrangement and design concept. It is desirable to use a variable-flow concept so that the large diversity often found in hydronic heating systems can permit the downsizing of convertors, pumps, and piping to minimize initial cost. Variable flow is easily obtained using VSDs on large systems and differential pressure control on smaller ones.
Hydronic heating water systems designed specifically for preheat coils should have a minimum 40 percent propylene glycol solution for freezer protection of coils. All outdoor air units should also have duplex coil circulating pumps to provide a continuously uniform heating water flow across the entire coil face areas. Coil circulating pumps may be either inline or base mounted and located for easy service. In-line pumps may be located overhead provided that safe service platforms and permanent rigging devices are installed to accommodate replacement of pumps. In-line pumps should not exceed 5 600 W in size.
Hydronic heating water systems should be segregated when different design temperatures are required because of seasonal changes. Those systems that serve reheat coils, perimeter radiation, and fan coil units may have the discharge temperature reset as a function of ambient conditions for improved operating efficiency.
For reheat and miscellaneous heating applications, such as hot water coils (duct mounted, furnished with air terminal unit, fan coil units), unit heaters, radiant panels, cabinet unit heaters, convertors, and so on, one common heating system should be provided. The use of two totally separate and independent heating systems (one for reheat and the other for perimeter terminal units) should be considered only if the use of two systems is proven costeffective by the life-cycle cost analysis. With a single, common heating system, care should be taken to select the lowest hot water temperature reset schedule to offset the generally constant reheat load of the interior spaces.
Heating elements in building heating systems should be connected in parallel. Where elements are utilized in conjunction with the cooling system supply, controls should be included with provision for adjustable dead band to avoid simultaneous heating and cooling, unless relative humidity control is essential, in which case simultaneous cooling and heating may be considered.
Piping for hydronic systems consists of Type K copper or carbon steel piping with threaded fittings for sizes 50 mm and smaller and Schedule 40 black steel for sizes 65 mm and larger. Heating piping should be sized per the following criteria:
- Copper pipe: 1.2 m per 30 m maximum head loss
- Steel pipe: 1.2 m per 30 m maximum head loss and 2.4 m/s maximum pipe velocity
Piping for heating water systems is normally extensive and routed to the remote areas of all buildings. During the schematic design phase, the project engineer should develop a piping concept that reduces long- and small-sized terminal runs and accommodates easy system balancing to all heating devices. A balancing valve and flow meter should be provided at the main branch from a riser at each floor for all systems. Wherever possible, reverse return piping networks should be employed, as they tend to be self-balancing and force flow to the remote ends of the network.
Each piece of heating equipment should have a means to balance the water flow, determine the water flow, and control the heat capacity. Large-capacity coils, over 0.63 L/s flow, shall have pipe-mounted flow meter and temperature and pressure fittings. Smaller coils should have pressure/temperature plugs on the supply and return mains to accommodate balancing.
Primary distribution pumps are always base-mounted, end-suction or split-case, doublesuction pumps. Close-coupled pumps are not acceptable. All pumps, coil circulating, and distribution should have the following components to support maintenance operations:
- Isolation valves on suction and discharge lines
- Pipe strainer or suction diffuser with strainer
- Flexible connections on suction and discharge
- Check valve on discharge
- Balancing valve with memory stop on discharge
- Pressure gauges and thermometers on suction and discharge lines
- Pump inertia base and vibration isolation when not installed slab on grade
- Pump speed not exceeding 26 rad/s
top
F.16 Fume Hoods and Biological Safety Cabinets
NIH fume hood and biological safety cabinet specifications should govern the requirements for specific types of hoods and cabinets. The A/E, after thorough review of program requirements, should select hoods on cabinets that will meet the needs of the researcher while optimizing system performance and minimizing energy cost. Current specification sections include the following:
- Section 11810: Fume Hood, Laboratory, Air Bypass Type
- Section 11820: Fume Hood, Laboratory and Horizontal Air Bypass Type
- Section 11830: Fume Hood, Laboratory and Combination Sash Type
- Section 15951: Testing Constant Volume Fume Hoods
- Section 15952: Testing Variable Air Volume Fume Hoods
- Section 15907: Testing Adjusting and Balancing
The type of hoods employed for use at the NIH should be based on a comprehensive lifecycle cost analysis that accounts for all system features required by the Division of Safety.
Variable or constant-volume hoods may be considered, but the health and safety of building occupants must not be compromised under either option. The use of variable air volume (VAV) fume hoods is highly recommended. The ongoing maintenance cost of any option should be considered in cost analysis.
Hazardous experiments involving toxic chemicals and/or unpleasant odors must be conducted within a chemical fume hood. Use of a chemical fume hood allows toxic materials and dust to go into the laboratory building exhaust system and be discharged to the outside.
A minimum system capacity is one 1 200 mm chemical fume hood (nominal 3.54 L/s) for every other laboratory module. Distribution capacity should be one 1 200 mm chemical fume hood per laboratory module. The Program of Requirements should govern whether the requirements are over this limit.
The NIH Division of Safety shall be consulted to ensure that it has discussed the needs of the requestor and has determined the best containment device (fume hood, canopy hood, slot hood, trunk exhaust, downdraft table, etc.) for the research effort. All specialty-made or shop-fabricated canopy hoods, slot hoods, sprinkle exhaust, and so on should be designed and constructed to the latest edition of industrial ventilation.
Auxiliary air-type laboratory hoods should not be used in new laboratory facilities. These hoods should be considered only in the replacement of existing auxiliary air fume hoods and then only if they are continuously monitored with respect to air balancing.
In any fume hood retrofit application for existing facilities, the A/E firm should verify existing system capacities inclusive of the auxiliary air and laboratory supply and exhaust system characteristics. Once it has been assessed in coordination with the Resource Management Section that the system(s) can support the addition or replacement of an existing bypass hood or auxiliary air hood, this information should be documented and the design allowed to progress.
Note that auxiliary air hoods discharge untreated or partially treated air just in front of the face of the hood (usually at head level), and a scientist working at the hood must work in unconditioned air. Partial heating of the air supply in the winter reduces the economical advantage of this type of hood. If improperly balanced, some of the auxiliary air may not enter the hood but will enter the air-conditioned space of the laboratory, sweeping with it some of the contaminated air from the hood, which is an undesirable condition.
The laboratory air supply distribution system should be sensitive to air motion within the laboratory space. Fume hoods rely primarily on face velocity through the hood’s face to provide a safe working environment for the researcher.
To optimize the performance of the hoods, they need to be located away from interfering drafts, airflow disturbances, supply and exhaust, and pressure differentials created by the swing of doors. Personnel circulation characteristics in front of the hood’s face should be minimized.
New and existing chemical fume hoods shall have as design criteria a face velocity of 0.51 ± 0.10 m/s with a uniform face velocity profile of ±10 percent of average velocity with the vertical sash fully open.
The A/E must realize in the early design phase that proper hood performance can be achieved only if the equipment is properly installed, the system is connected to a properly designed exhaust system, the supply system is capable of delivering the hood ventilation requirements, and the location of the hood has been properly selected within the laboratory area. For correct positioning of the laboratory hood, the designer must follow the design methodologies detailed in the NIH publication Methodology for Optimization of Laboratory Hood Containment to evaluate the likely hood containment performance.
Improper assessment of any of these factors will result in poor hood performance. Fume hoods and biological safety cabinets at the NIH are used continuously and should be “on” 24 hours per day, 7 days per week. In addition, there should not be user control or nighttime setback control capability.
F.16.1 Variable Air Volume Hoods: VAV hoods may be considered for use in NIH buildings provided the following issues are evaluated and requirements met:
- The hood must meet current fume hood specifications, with the exception that they are not the bypass type. However, partial bypass may be needed to meet minimum exhaust requirements for the lab to maintain face velocity at 150 mm sash height.
- The hood should have no air-cleaning (HEPA or charcoal) or stack-sampling devices in the exhaust system (stack sampling is done on high-level-radiation hoods; biological cabinets have HEPA filtration). Varying the volume may interfere with the operation of this equipment or the current monitoring techniques used.
- The hood should have no other auxiliary equipment (high-velocity/low-volume exhaust systems) on the same exhaust fan. This type of equipment is generally designed for a fixed volume and may not function properly if the volume of flow is decreased.
- The laboratory must remain under negative pressure with respect to the corridor or adjoining rooms even at the minimum exhaust rate, if negative pressure was part of the original laboratory design. When the exhaust quantity is reduced, the supply quantity must be reduced by the same volume.
- The laboratory must maintain minimum required air changes per hour (six) with hood(s) in the minimum exhaust rate position.
- There should be no extenuating circumstances based on hood and/or laboratory use that preclude the use of VAV systems. Examples of these circumstances might be (1) odorous compounds used on the lab bench and (2) excessive heat generation in the laboratory from process equipment or high-release compounds that require full exhaust rate dilution. These conditions might produce hazardous conditions if the exhaust volume is reduced.
- An airflow monitoring/alarm device must be installed at the hood to provide operating information to the hood user. The methods that are to be evaluated for monitoring the airflow include (1) velocity sensing in the hood side wall, (2) sash position determination, and (3) pressure sensing between hood and room.
- An override capability must be provided to allow the user to have maximum exhaust regardless of sash position.
- It is important for the hood user to know whether the hood is a variable air volume hood and to understand how it operates.
- Control response time and stability should be reviewed to provide consistent repeatable performance.
- A constant face velocity should be maintained at the fume hood sash at varying sash openings. The following methodology should be evaluated to achieve the constant face velocity: (1) velocity measurement in the ductwork, (2) velocity measurement of the hood in the annular space between the outside and inside casing, and (3) direct sash position measurement. The analysis should consider the following factors. First, the measurement of velocity pressure is difficult in the exhaust ductwork. The air velocity typically is very low, with correspondingly low velocity pressure. In addition, the devices may become corroded if the exhaust stream contains material that will attack the sensor. Second, air velocity measurements in the annular space are sensitive to changes in airflow patterns in the room as well as in the hood. Third, an option is to vary the sash position, which could be connected by a cable to a potentiometer or other control system that transmits a signal to the volume control, which opens or closes the damper.
F.16.2 Fume Hood Testing and Alarms System: Fume hoods in new laboratory facilities should have a pressure-independent flow-monitoring device connected to a local audiovisual alarm within the laboratory area. For existing facilities, the implementation of airflow devices for fume hoods occurs during the renovation phase. When the fume exhaust falls below a preset safety level, the alarm will sound and the alarm light will come on.
All parts that are to be in contact with vapors/fumes in the hood, i.e., the sensing device, wiring, and so on, should be chemically resistant. All alarm systems should be UL approved. There should be a means to shut off the audible alarm to reset. The alarm should have an internal timer so that the audible alarm is reactivated after a specified time (adjustable between 5 minutes and 15 minutes). The alarm should have the capability to set the controller’s setpoint to the safety level desired. There should be a means for setting the controller’s setpoint to the exhaust level desired. This adjustment shall be “internal” so that it is not readily adjustable by operating personnel. Upon return to normal flow, the alarm should sound again until reset.
The ACGIH Guidelines are referenced in the guidelines for fume hood testing. The ACGIH requirements do not specifically address all testing issues required by the NIH. The following criteria shall be used for testing fume hoods in NIH buildings:
The fume hood manufacturer, no later than 30 days after receipt of the order, shall provide to the owner the use of a state-of-the-art fume hood test facility meeting the requirements of the latest SMACNA Standard LF 10.
The hood manufacturer shall conduct modified ASHRAE Standard 110, 1995, protocol of 1 800 mm hood of similar design to the type specified. The bypass shall be designed so that face velocity does not exceed the maximum as the sash is lowered in a variable volume hood. Variable volume fume hood protocol of 1 800 or 1 200 mm shall be tested in accordance with the Modified ASHRAE 110 Test for minimum baseline requirements. The manufacturer shall provide a fume hood control system at its state-of-the-art test facility meeting the requirements of the latest SMACNA standard LF 10 on its cost for acceptance by the NIH prior to the delivery of hoods for installation. The minimum of 50 percent installed hoods at site will be again offered for testing on site by the contractor after installation and building balance prior to occupancy. The contractor shall arrange for tests to be conducted by an NIH-approved independent testing contractor. The specifications should clearly identify the type of measurement devices that test a constant face velocity, such as hot wire anemometer, heated thermocouple anemometer, impact tube and side wall or other static tap, pitot tube, and so on, or measure volume or mass flow rate using devices such as orifice and differential pressure measurement system, nozzle and differential pressure measurement system, turbine flow meter, swirl flow meter, and vortex shedding meter. A hood of design similar to the type specified in the ASHRAE Standard 110 will have the parameters described in the next paragraph.
F.16.3 Fume Hood Testing: Note: This item must be included in the balancing specifications, the fume hood control specifications, and the hood specifications.
F.16.3.1 Fume Hood Containment Testing (Onsite): Laboratory areas and variable volume fume hoods shall be tested as installed to assess the level of containment. The test identified below was created by Farhad Memarzadeh, Ph.D., P.E., of the National Institutes of Health in 1997 and revised by Memarzadeh and Brightbill in 1999 and shall be performed during static and dynamic conditions. Testing shall be conducted as outlined below for 50 percent of the hoods provided in the project. Tests shall be characterized and referred to in two basic categories, “Static” and “Dynamic.” While elements of both static and dynamic testing exist in both test categories, these names are generally used for reference.
F.16.3.2 Static Testing: Testing shall be conducted in accordance with ASHRAE 110 - Method of Testing Performance of Laboratory Fume Hoods with the following modifications. This is primarily a test of the hood and laboratory configuration.
Hoods will be tested with simulated apparatus. This apparatus will consist of two each 3.8 L round paint cans, one 300 x 300 x 300 mm cardboard box, and three each 150 x 150 x 300 mm cardboard boxes. These items will be positioned from 150 to 250 mm behind the sash, randomly distributed, and supported off the work surface by 50 by 50 mm blocks.
- The test gas will have a 6 L/min flow rate.
- Each test duration will be 5 minutes.
- Acceptable test results shall not exceed 0.05 ppm.
- At the conclusion of each 5 minute test, there will be three rapid walk-bys at 300 mm behind the mannikin. Each two walk-bys will be spaced 30 seconds apart. If there is a rise in test gas concentration, it cannot exceed 0.10 ppm and must return to 0.05 ppm within 15 seconds.
- There will be a minimum of three and a maximum of five persons in the test room during the test procedure.
- Representatives of the NIH will witness the tests.
F.16.3.3 Dynamic Testing: Dynamic testing primarily tests the dynamic performance of the fume hood control system. This group of tests measures hood performance parameters through various dynamic “events.” Events shall include four sash movements up and down across differing ranges: 25-100 percent and 50-100 percent, sash movements of other hoods on the exhaust duct, walk-bys in front of the hood, and opening and closing the laboratory door commensurate with a person entering and exiting the room. Hood parameters to be determined for each event are defined as follows (refer to Figure F.16.3.3 below for a graphical representation of some parameters):
- Measured Face Velocity (FVm expressed in m/s): Face velocity measured in the plane of the sash. Samples shall be recorded at no less than 10 Hz. Sensing methodology shall have an internal time coefficient of no more than 100 ms.
Definitions:
- The internal time constant (ITC) is the amount of time it takes the sensor to respond 63 percent of the way to a step change.
- The response time is the length of time to get to within the stated accuracy of the sensor.
- Response time = ITC x 3 or 5 depending on the accuracy. Example: If the response time is 200 ms, the ITC = 40-70 ms.
There shall be a point sensor located in the middle of the face opening when the sash is at the lowest position during the tested event. No fewer than three point sensors shall be used. Averages shall be calculated for any point in time to assess overall measured face velocity; however, individual sensor samples shall be used in calculating turbulence intensity (TI).
- Total Exhaust Airflow (TEF expressed in L/s): Total exhaust flow measured in the main exhaust duct leaving the hood. This parameter shall be recorded at no less that 10 Hz. The sensing methodology used for the recorded data shall represent the total airflow through the full range of flows and be validated by independent multipoint measurement. If the fume hood control system uses a flow-sensing element, that element may be used assuming it can be calibrated across the full range of flow. Sensing elements must have an internal time coefficient of no more than 20 ms.
- Variable Face Area (FAv expressed in meters): Face area of the hood that varies as the sash is moved within specified limits.
- Fixed Face Area (FAf expressed in meters): Face area of the hood with sash at minimum position (minimum position should correlate with the minimum bypass flow through the hood).
- Hood Airflow Leakage (HAL expressed in L/s): The difference in airflow between the measured airflow through the face (at minimum position) and the total airflow measured in the exhaust duct.
- Calculated Face Velocity (FVc): Face velocity determined from the following equation: (TEF-HAL x 1 000)/(FAv + FAf).
- Steady State Face Velocity (SSFV): The average of all sampled face velocities for a 5 second period. Two SSFVs will be determined for both measured face velocity and calculated face velocity; one before the event (SSFVb) and one after (SSFVa). The SSFVa will start 2 seconds after the end of TSS. The second suffix of m for measured and c for calculated shall be used to indicate the type of assessment.
- Face Velocity Baseline (FVBL): The average of SSFVa and SSFVb.
- Control Linearity (CL expressed in %): Abs (SSFVa-SSFVb)/(FVBL) x 100.
- Time to Steady State (TSS10 and TSS5 expressed in seconds): The elapsed time from the initial sash movement until the FVc reaches and stays within ±10 percent or ±5 percent of FVBL (as indicated by the subscript).
- Face Velocity Overshoot/Maximum Deviation (FVO expressed in percent): Calculated using the Calculated Face Velocity sample farthest from the FVBL (FVf) throughout the test per the following equation: (Abs (FVf-FVBL)/FVBL) x 100. Samples include initial face velocity deviation immediately following the sash movement as the controls initially respond to the movement of the sash.
- Response Time Constant (RTC expressed in seconds): Elapsed time between initial movement of the sash and the initial subsequent movement of the exhaust valve.
- Steady State Deviation (SSD expressed in %): Face velocity variation from SSFVa or SSFVb as applicable. Calculated using the farthest sample from the applicable SSFV (FVf) using the following equation: (Abs (FVf-SSFVx)/SSFVx) x 100.
- Controllability (expressed in mV/mm): Describes controller response to changing sash position, i.e., controller’s response signal change per unit distance of sash movement.
- Sash Position (SP expressed in mm): For vertical sashes, vertical distance from the sill of the hood to the bottom of the sash. The minimum sash position shall correlate with the position of the sash when the minimum flow through the hood is all through the face. Maximum sash position shall be defined as a distance of 550 to 650 mm. This parameter shall be recorded at no less than 10 Hz.
- Controller Output (CO expressed in volts): Control output to the controlling exhaust air valve. This parameter shall be measured and recorded at no less than 10 Hz.
- Turbulence Intensity (TI expressed in m/s): Calculated root mean square of the fluctuating face velocity determined using FVm. This value shall be calculated for each of the steady state conditions preceding and following each event. This shall be correlated with a “box leakage factor” of the installation using the Methodology for Optimization of Laboratory Hood Containment (MOLHC) by NIH Office of Research Services, Farhad Memarzadeh, Ph.D., P.E., principal investigator. While this value does not have a pass/fail requirement, it is the fundamental indicator of containment and therefore shall be clearly reported.
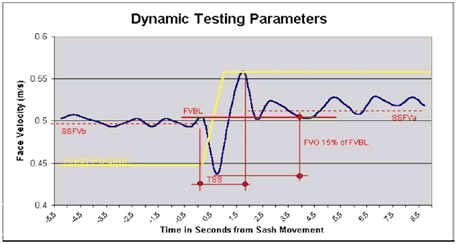
Figure F.16.3.3 Dynamic Testing Parameters
F.16.3.4 Parameter Performance
Parameter Performance Requirements:
- Face Velocity Baseline (FVBL): 0.51 m/s ± .05 m/s
- Control Linearity (Cl expressed in %): < 2%
- Time to Steady State10 (TSS10 expressed in seconds): <2 seconds
- Time to Steady State5 (TSS5 expressed in seconds): <3 seconds
- Face Velocity Overshoot/Maximum Deviation: <15%, which means at no point throughout the test shall a sample be recorded <0.43 m/s or >0.59 m/s
- Response Time Constant (RTC expressed in seconds): <0.5 seconds
- Steady State Deviation (SSD expressed in %): <5% assessed using calculated face velocities
- Controllability (expressed in mV/mm): >12 mV/25.4 mm
F.16.3.4.1 Alternate Parameter Performance Requirements: The following performance parameters are alternate requirements that can be used in assessing acceptable dynamic responses:
- Face Velocity Baseline (FVBL): 0.51 m/s ± .05 m/s
- Calculated Face Velocity (FVc): All samples >0.255 m/s and <0.89 m/s, meaning that at no time during the event shall the calculated face velocity be outside that range. Any sample recorded beyond that range will result in assessing the response as unacceptable.
- Control Linearity (Cl expressed in %): <2%
- Time to Steady State10 (TSS10 expressed in seconds): <1.6 seconds
- Time to Steady State5 (TSS5 expressed in seconds): <2 seconds
- Response Time Constant (RTC expressed in seconds): <0.5 seconds
- Steady State Deviation (SSD expressed in %): <5% assessed using calculated face velocities
- Controllability (expressed in mV/mm): >12 mV/25.4 mm
- Test Execution: Testing agency shall be equipped to execute the testing and assess all performance parameters on site the day of the test. Data acquisition of required parameters shall be simultaneous.
- Test Documentation: All testing, calculated, and recorded parameters shall be presented in a report that shows the recorded parameters graphically and tabulates and summarizes all the results. Performance of the hood, the hood controls, and the laboratory in general shall be described and summarized.
Note: Fume Hood Control Testing (Offsite-Mockup) must be included only in the control manufacturer’s specifications.
F.16.3.5 Fume Hood Control Testing (Offsite-Mockup): The manufacturer of the proposed fume hood control system shall mock up a fume hood installation and demonstrate the performance of its system to validate that they can meet the requirements specified herein. The offsite test shall include all parameters under the control of the control system (FVBL, TSS, CL, RTC, SSD, and Controllability). It is not necessary to mock up the installation and assess TI. Events to be tested off site include all specified sash movements on the hood being tested. Walk-by and door-opening affects are not required for the offsite test.
The testing shall be accomplished by an independent testing agency approved by the A/E and the NIH. Reports shall be provided with the laboratory control submittals, and no approval will be given for the fume hood control system until documentation of successful demonstration of the performance requirements is submitted.